Pain
To understand better how pain can be managed, it is helpful to understand the mechanisms and processing of pain signals and sensations within the body. This is regulated by the nervous system, with the pain signal being carried by nerve cells called neurons to the body’s signal processing centres, generally referred to as the central nervous system (the spinal cord and the brain).
There are two different types of pain, both following different mechanisms of pain sensation. Acute pain follows a well understood mechanism and can be predicted and managed with relative ease. The mechanisms of chronic pain are less well understood and so chronic pain can be more difficult to manage.
Below we provide a brief view of the important parts, steps and mechanisms in pain signalling. We think that this is important for understanding how pain is managed (for example, in local or general anaesthesia), where these mechanisms can go wrong and, for example, may result in chronic pain, neuropathies, or a range of facial pain syndromes.
Mechanics, neurons and general principles
Pain signals follow a general mechanistic pathway through the body from the source to the processing centres (Figure 1).
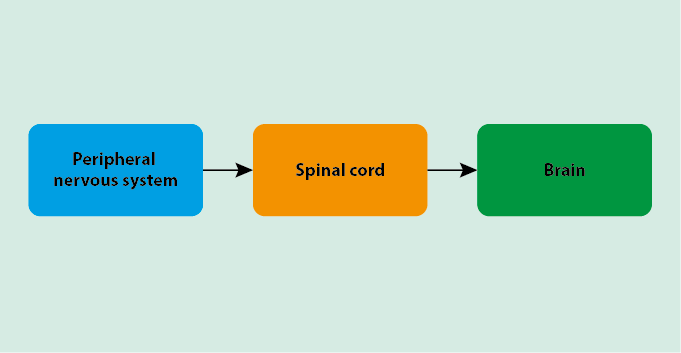
When a stimulus is applied, specialised nerve cells (neurons) in the peripheral nervous system detect the signal and propagate it through the spinal cord to the brain. The spinal cord and brain process the information as part of the functions of the central nervous system, causing the actual pain sensation.
Neurons are found throughout the body. Their structures are well adapted to detect stimuli and transfer the resulting signal through the body (Figure 2).
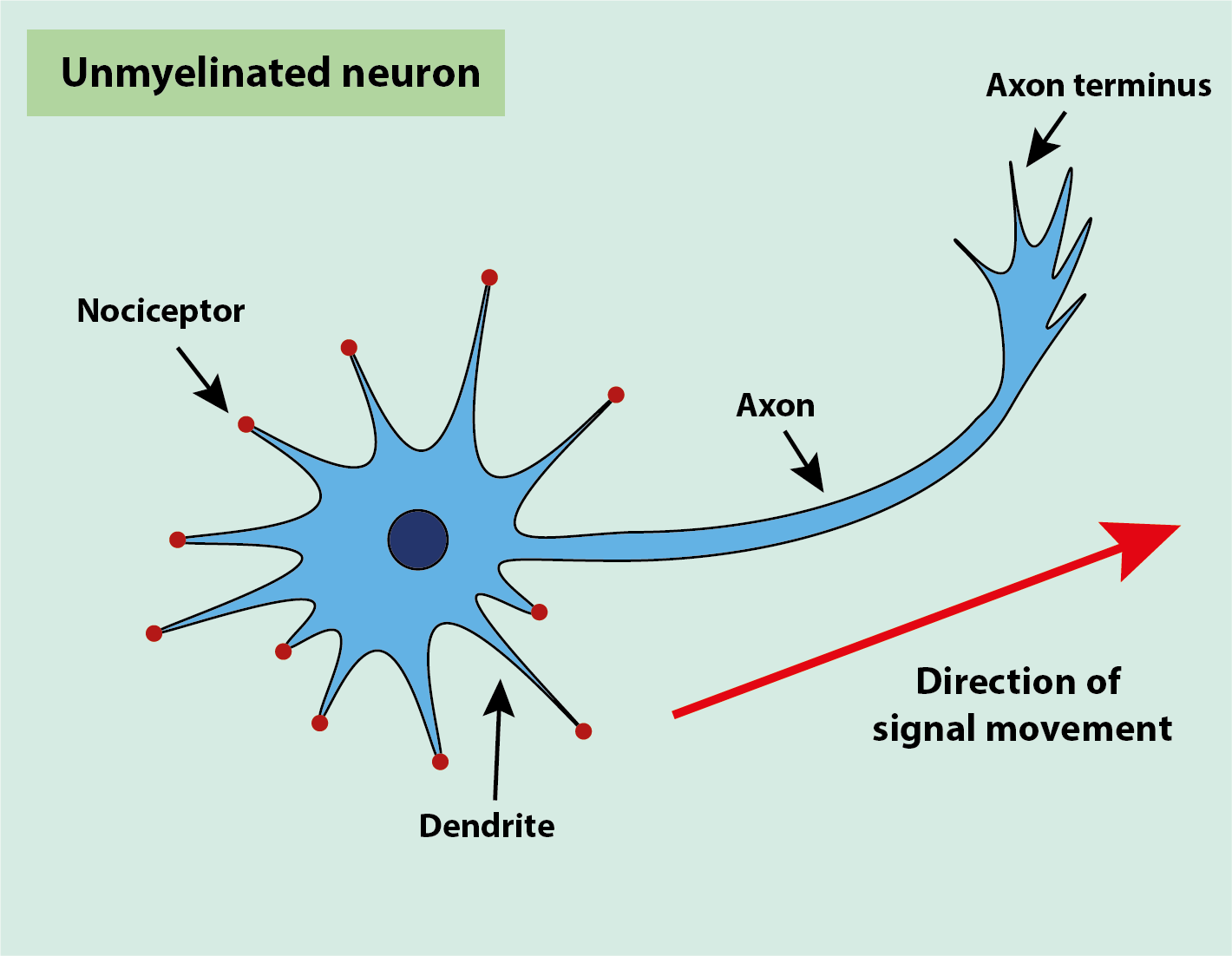
Nociceptors are specialised sensory detectors on free nerve endings, dendrites, which detect noxious (unpleasant) stimuli. These stimuli are converted to electrical signals by the nociceptors. The (minor) energy input from the stimulus alters the membrane permeability, allowing small ions, such as Na+ ions, to permeate the membrane more easily. This produces a tiny depolarisation on the interior of the dendrite, which is the primary electrical event. The electrical signals are then passed down the axon to the axon terminus, and on to the next neuron unit
Neurons are classed as excitable cells. All excitable cells have a negative voltage inside the cell compared to the voltage outside the cell (Figure 3). This voltage is established by:
- potassium ions, K+, moving out of the cell through the cell membrane via non-gated (not controlled and happening all the time) K+ ion channels along a K+ concentration gradient;
- sodium ions, Na+, being present outside the cell but not being able to move in through the membrane as its gated (controlled) transport channels are closed.
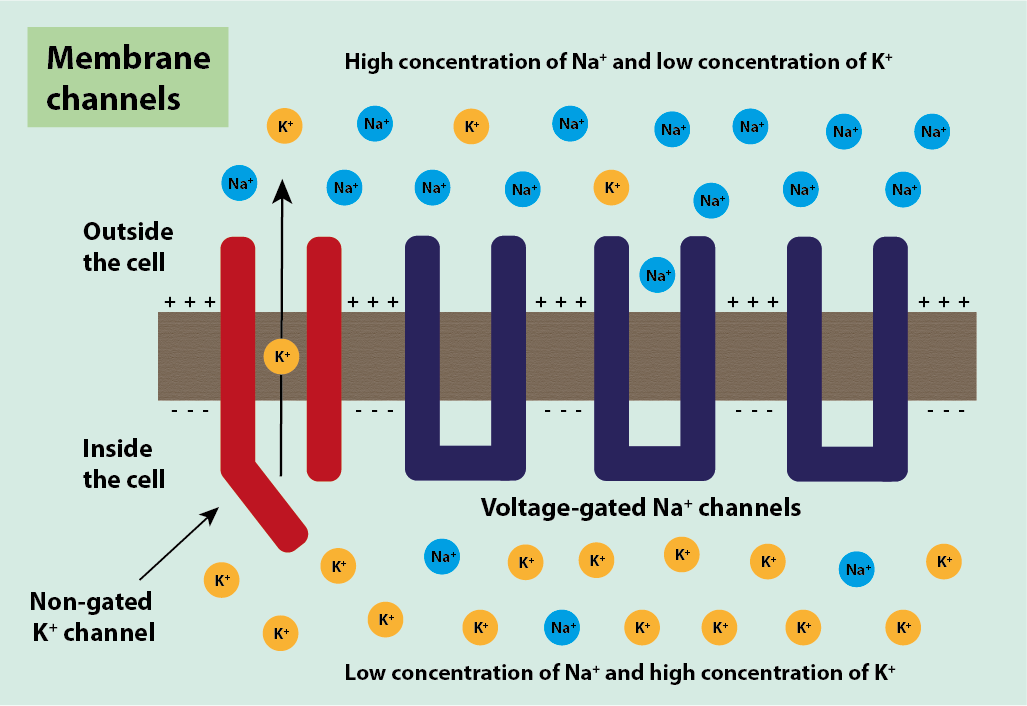
In the body, the different ions tend to be spread out evenly. As a consequence, ions will move from an area of high concentration to low concentration, along the concentration gradient, without any stimulus being required. This is similar to the increasingly even distribution over time of sugar in a hot drink, even if the brew is not stirred. Initially, there will be a higher sugar concentration where it was added, over time the dissolved sugar molecules will spread throughout the liquid and eventually will be evenly distributed. The same principles of dissolution of electrolytes applies in the body (which for this aspect may be seen as behaving like a liquid; not an unreasonable view given that a body consists of approximately 80 percent water). Gradients are used in the ion channels passing through cell membranes where ions move from a high concentration to a low concentration area without stimulus.
With K+ ions moving out of the cell constantly through non-gated K+ ion channels and no positive ions moving in under normal conditions, overall this leaves the inside of the cell with a lack of positive ions compared to the outside of the cell. There are also voltage-gated K+ ion channels in the cell, which can be used to move K+ ions faster out of the cell; the voltage (charge) of the cell controls these gated channels.
The voltage-gated Na+ ion channels work in a similar way to the voltage-gated K+ channels and are also controlled by the voltage of the cell. They contain a voltage-sensing region that is positive and is attracted to the negative region either inside or outside the cell. In normal conditions, it is attracted to the negative inside of the cell and sits towards the inside of the cell causing the channel to be closed (Figure 4).
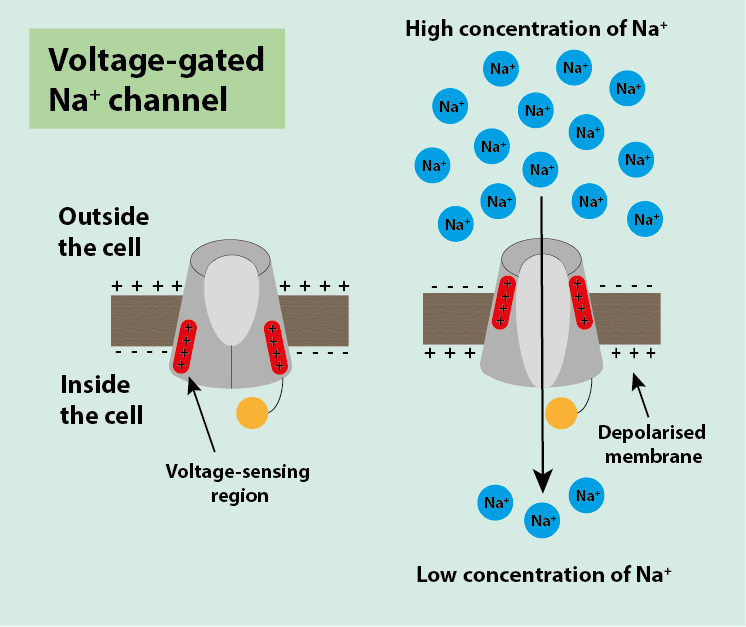
Neurons harness these gradients of ions when it comes to propagating a signal along its length. When a signal is detected by the nociceptors of the neuron, the neuron undergoes a small depolarisation. Over this depolarisation, the inside of the cell becomes more positively charged than the outside of the cell, the opposite of how it was before. As this depolarisation makes the outside of the cell more negative, the voltage-sensing regions in the voltage-gated Na+ ion channels move towards the outside of the cell. This causes a conformational change in the channel, opening it and allowing Na+ ions to move into the cell through the channel. This movement makes the inside of the cell more positive, increasing the depolarisation and passing it along to the next section of the axon where there are more closed voltage-gated Na+ ion channels. These channels react in the same way to the depolarisation and the signal is thus passed along the axon (Figure 5).
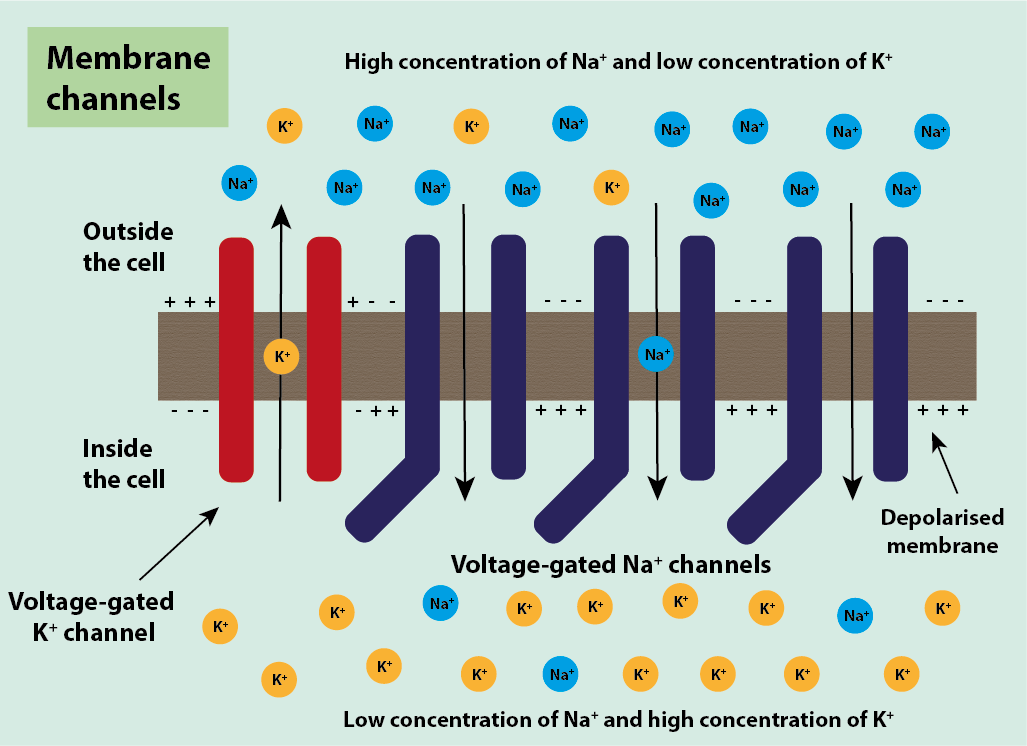
The voltage-gated Na+ ion channels have a segment called the channel-inactivating segment, which, once the channel has been opened, moves into the open channel to inactivate it and stop more / too many Na+ ions entering the cell (Figure 6).
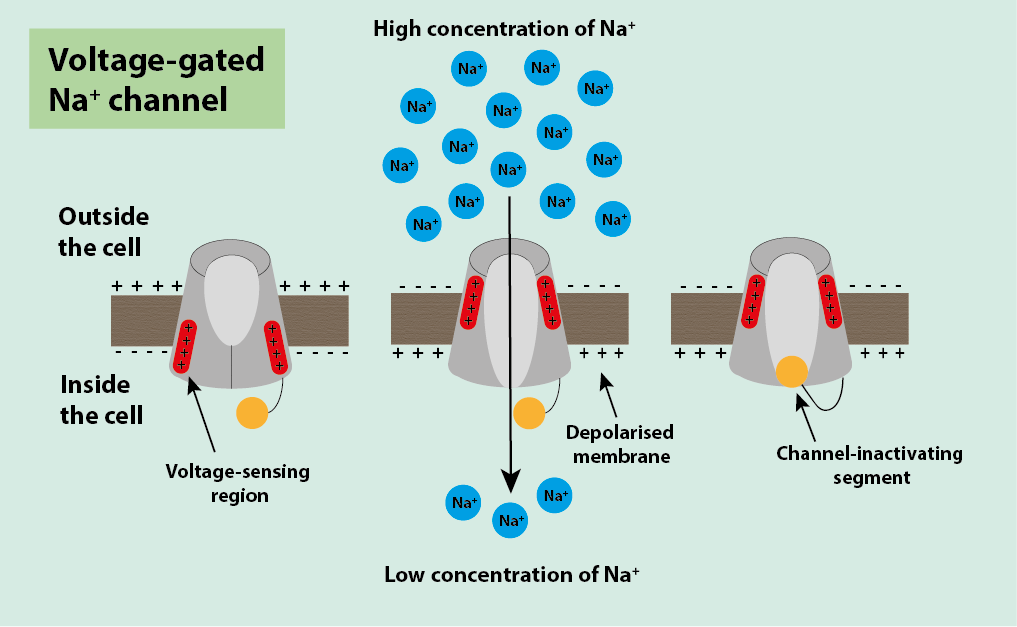
The inactivating segment remains in place while that part of the membrane is still depolarised, stopping more signals being sent through that part of the neuron. This allows distinction of different signals, and attenuation of each signal to give the correct level of response for the stimulus. The duration of signal transmission is the same, only the number of signals changes to indicate the level of pain.
The cell voltage must be reset, back to normal after the depolarisation. This is achieved through the voltage-gated K+ ion channels, which open when the inside of the cell is positively charged, allowing more K+ ions to be removed from the cell. This removes the excess of positive charge from the inside of the cell, restoring the normal voltage of the cell.
An unmyelinated neuron (see Figure 2) propagates the signal in the way described above, with a signal passing through an axon at speeds of up to 1 m/s. Unmyelinated neurons are typically C fibres (see Table 1) and are generally responsible for the detection of temperature changes, such as noxious heat or cold. Complex movements, such as those needed to run, require much faster signal propagation. This is achieved through myelination of the neurons. Myelination is the partial covering of the length of the neuron with a lipid (a fatty acid) called myelin. There are gaps in the myelination called the nodes of Ranvier where no myelin is present and where the transmitted signal can ‘jump along’. The myelin sheath acts as insulation on the neuron and increases the rate of signal transmission along the neuron (Figure 7).
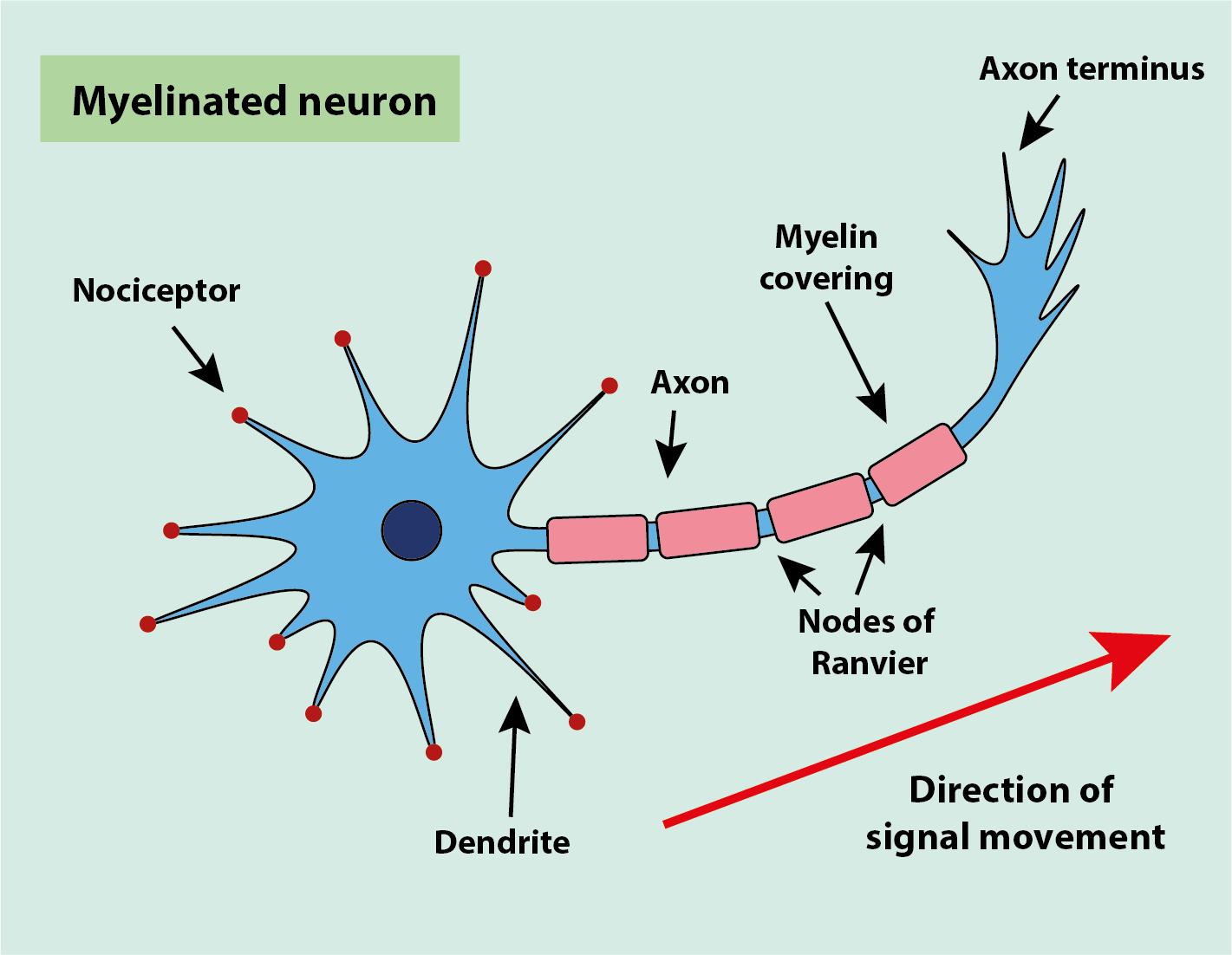
The myelin sheath prevents any movement of ions in or out of the cell. Only in the regions not covered by myelin, the nodes of Ranvier, can ions move in or out of the cell. All of the voltage-gated channels are located in these nodes in such neurons. This speeds up propagation of the signal, as depolarisation at one node causes an excess of positive ions in that region. That surplus diffuses down the axon rapidly to the next node causing depolarisation there. This allows the signal to effectively jump along the axon, causing it to conduct the signal at speeds of 10 to 100 m/s, much faster than the unmyelinated neurons conduct. The nodes of Ranvier and the ‘jump along’ mechanism are relevant in the context of effective local anaesthetic nerve blocks and the underlying mechanism of blocking pain signals from spreading at these locations.
There is a threshold the signal must surpass for it to cause a depolarisation in neurons and to be detected. This is required to make sure that small fluctuations in the voltage do not cause a signal for pain. The activation threshold is different for each fibre and is based on what they are meant to detect. Only a small stimulus is needed for non-noxious signals. These are sensations that are felt but not painful, such as the light touch of someone putting their hand on your shoulder. A large activation threshold is needed for fibres that detect more serious pain to ensure they are not stimulated by anything other than the serious pain they are meant to detect.
Afferent fibres are the nerve fibres that carry a pain/sensation-inducing signal towards the central nervous system. Of these afferent fibres, there are three main classes of fibres detecting stimuli:
- Aβ fibres;
- Aδ fibres;
- C fibres.
Each of these different classes detects different types of stimuli.
Aβ fibres differ from the other two classes, as they are the only class that detects non-noxious stimuli (Aδ fibres and C fibres detect noxious stimuli). Aβ fibres respond to light touch and do not create a pain signal upon stimulation, so are not relevant in transmitting pain signals. They are included in Table 1 (below) as a comparison to the pain detecting fibres.
Table 1 Properties of the different classes of afferent nerve fibres
Aβ fibres | Aδ fibres | C fibres | |
---|---|---|---|
diameter | large | small | very small |
myelination | high | slight | none |
speed of signal conduction | rapid | slow | very slow |
level of stimulus required | low | both low and high | high |
responsive to type of stimulus | non-noxious | noxious | noxious |
response to stimulus | no pain signal | rapid, sharp, localised pain | slow, burning, dull pain |
There are three different general classes of stimuli that cause a pain sensation:
- mechanical;
- thermal;
- chemical.
Different afferent neuron fibres respond to different stimuli, with Aδ fibres responding to mechanical and thermal stimuli, and C fibres responding to all three classes.
The greater the degree of myelination of a nerve fibre, the faster is its speed of signal conduction; Aδ fibres conduct pain signals faster than C fibres. C fibres have a larger activation threshold, as stimulation of these fibres causes more serious pain, so it needs to be ensured that C fibres are not stimulated by any irrelevant stimuli.
Once the signal reaches the end of the axon, it reaches the synapses to the dendrites of other neurons to pass on the signal. Synapses are the gaps between the neurons where the signal must pass through. The signal has been conducted electrically in the neurons, however, to cross the synapse, it becomes a chemical signal. The electrical signal stimulates the release of the chemical messenger molecules. There are vesicles in the axons containing these chemical messenger compounds, known as neurotransmitters.
Neurotransmitters are small, water-soluble molecules (such as acetylcholine, L-glutamate or dopamine), which can diffuse around the body or, in this case, across a synapse. There are specific receptors for each neurotransmitter, with binding causing localised charge changes in the neighbouring neuron, turning the signal back to electrical and thus propagating the signal along the nerve fibre. The receptors are specific for a particular neurotransmitter (the key and lock principle of mutual fit). When the electrical signal reaches the end of the axon, the vesicles undergo exocytosis (they burst), releasing the neurotransmitter molecules into the synapse (Figure 8). The neurotransmitters diffuse across the synapse very quickly and bind to the receptors on the dendrites of the next neuron.
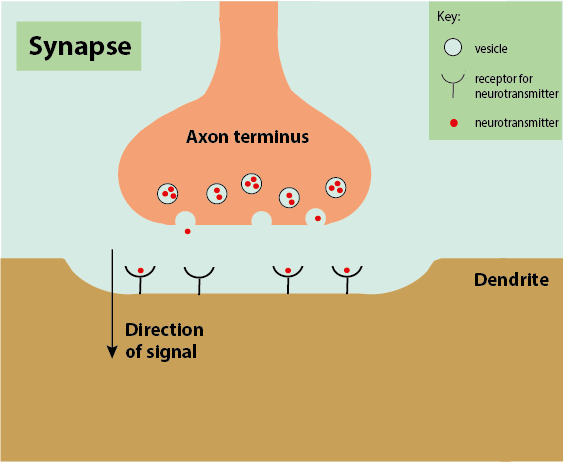
Neurotransmitters are chemical signals in many different processes. While they conduct the pain signal in synapses, they can also disrupt or block the travel of a pain signal. For example, this can occur by different neurotransmitter molecules blocking a receptor site. This principle can be used to modulate pain.
The neurons in the peripheral nervous system synapse to neurons in the spinal cord, passing the signal to the central nervous system where it is further processed (Figure 9).
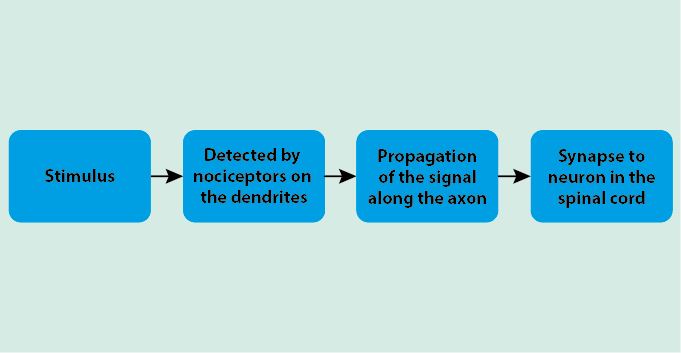
Once the neurons from the peripheral nervous system synapse to the neurons in the spinal cord, the signal is propagated up the spinal cord and to the brain’s processing centres. The signals commonly pass through three areas of the brain. First, the signal goes through the brain stem, known as the medulla, which connects the spinal cord to the brain. From here, the neuron from the medulla synapses to neurons in the thalamus of the brain, which is a sensory interpretation area of the brain, passing on the signal. Finally, the thalamus synapses to the somatosensory cortex of the brain, which are the particular regions of the brain that specialise in processing pain.
The pain-processing areas in the brain can interpret the incoming signals and cause the corresponding pain sensation to be felt elsewhere in the body (Figure 10).
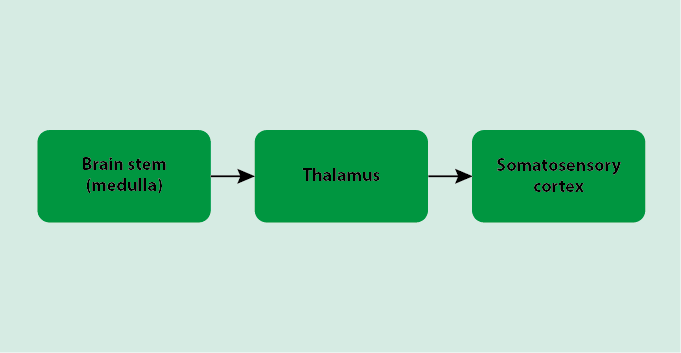
The general pathway for pain sensation, following the route from peripheral nervous system, to the spinal cord and then to the brain, is known as the spinothalamic tract (Figure 11). There is another tract known as the spinoreticular tract. However, that tract is more involved in the emotional aspects of pain than the sensation itself.
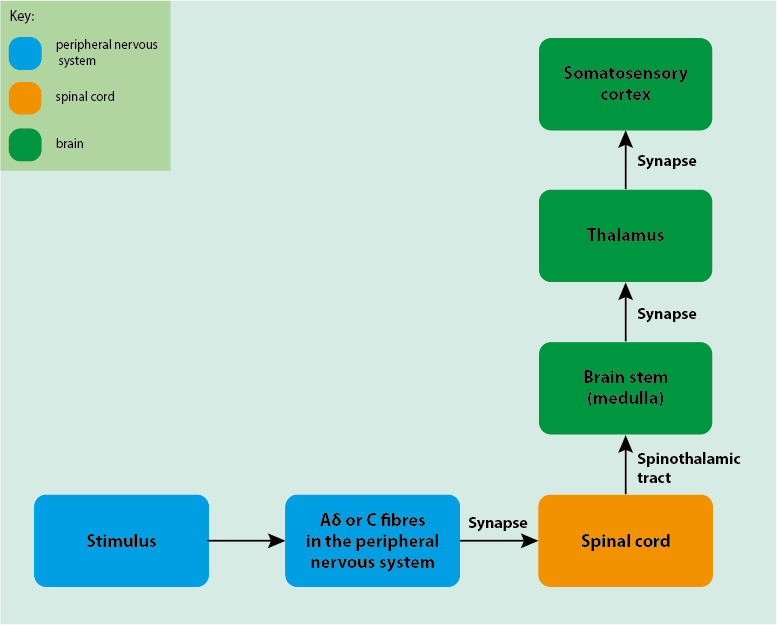
The body has its own mechanisms of inhibiting pain. These can sometimes be involved in pain modulation. One well-known example is the scenario of bumping one’s head. When we bump our head, we feel pain, but when we rub it, it feels better. This is due to activation of the Aβ fibres, which detect the rubbing as a non-noxious stimulus. This stimulates inhibitory interneurons in the spinal cord, which have a blocking effect, inhibiting (some of) the pain signal from the C fibres by hyperpolarising the neuron that would have passed the pain signal to the brain (Figure 12).
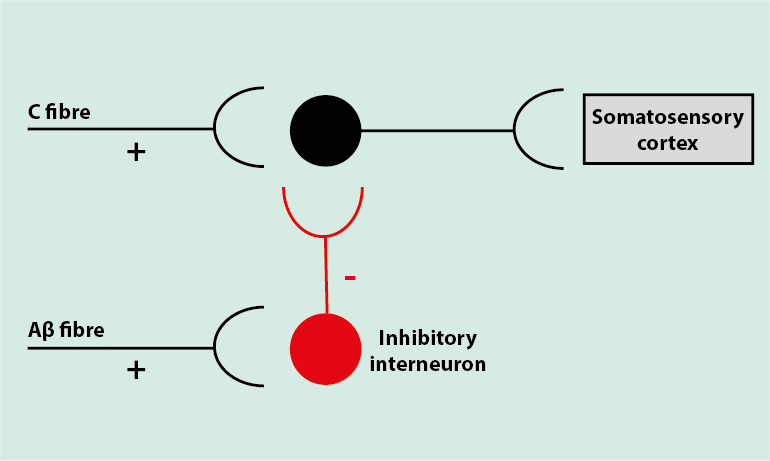
This blocking effect is due to neurotransmitters in the synapse between the inhibitory interneuron and the neuron in the spinal cord that takes information to the brain (to the somatosensory cortex). The inhibitory interneuron releases neurotransmitters GABA and glycine, rather than the usual neurotransmitters. These ‘wrong’ neurotransmitters interrupt the pain signalling to some extent.
Another way the body naturally inhibits pain is via descending pathways. These occur after stimulation of the grey matter in the brain and descend from the brain stem to the spinal cord, having an analgesic effect. This is thought to be due to the release of natural opioid-like substances, known as endogenous opioids, (which act as analgesics). Endogenous opioids, such as β-endorphins and enkephalins, bind to µ-opioid receptors on neurons. This binding supresses the pain signal as these endogenous opioids act as inhibitors of excitatory neurotransmitters.
Acute pain
Acute pain occurs as and when needed, when the pain-signalling system is functioning correctly. It is a widely accepted view that acute pain is designed to protect the body from further damage. The mechanisms described above are at work to create this alert system and require the correct functioning of all the sub-systems to work correctly. With these mechanisms of pain sensation being well understood for acute pain, it is easier to manage as certain parts of the mechanism can be targeted to reduce or eliminate the pain sensation felt.
In general, acute pain mainly acts when an injury (or other lesion) occurs, and gradually ramps down during the healing phase. During the healing phase, its main protective role is to prevent over-usage of an injured part of the body. This can be a disadvantage when it stops the use of the area for too long and so may hamper recovery. If the mechanisms for acute pain sensation malfunction in some way(s), this is one potential origin for chronic pain.
The simple concept of acute pain as a warning mechanism becomes quite confused when one considers acute pain examples from the maxillofacial region:
- Toothache – 32 nerve and blood vessel complexes (in a fully dentate adult), completely surrounded by dense, non-expandable tissue. The only subjective cerebral response to stimulation of this complex is pain. The healing response of inflammation can quickly result in terminal ischaemia (inadequate blood supply) of the complex, rendering it highly susceptible to subsequent infection. It is quite difficult to see the evolutionary advantage to acute pain in this context.
- Oral ulceration – oddly, benign mucosal ulceration is often temporarily exquisitely painful, whereas the ulceration of oral cancer is often not painful. The evolutionary advantage of acute pain here is almost oxymoronic.
- Fractures of the jaws – here the acute pain is functioning as it should. Displacement and movement of the fractured bone ends are painful. Restoration of correct position (known as reduction) and fixation to prevent movement provides good pain relief and the optimal healing environment.
- Trigeminal neuralgia – effectively a sensory form of epilepsy, giving rise to extreme acute pain of absolutely no benefit to the sufferer. This can have a chronic pre- or post-neuralgic type of pain but in its commonest form is simply acute pain in a pure but entirely dysfunctional form.
Chronic pain
Chronic pain has no protective role and it occurs when the pain-signalling system is malfunctioning. There are many different ways the system can malfunction. There is no useful function to chronic pain, with it simply causing pain when there should not be any. Because of this, chronic pain is also sometimes referred to as neuropathic pain and may be considered a disease in its own right.
There are three main areas where pain-signalling malfunction can occur:
- the peripheral nervous system;
- the transmitting nerve fibres;
- the processing of pain signal in the brain.
Table 2 summarises some of the main categories and types of chronic pain. These most common examples of sub-types of chronic pain are given clinical names. There are some common causes of failure for these pain syndromes. All of these malfunctions can be categorised as inhibition malfunctions, where the system fails to stop pain signals being sent at the wrong time, for too long, or from the wrong place.
Table 2 Some sub-types of chronic pain
Subtypes of chronic pain | Normal inhibition | Failure of inhibition |
---|---|---|
Hyperalgesia (heightened response to a usually mildly painful stimulus) | Inhibition of the neurons before and after the synapse by inhibitory interneurons and descending inhibitory pathways to attenuate the signal and give the correct level of response for the stimulus. | Inhibition does not occur, probably due to inhibitors such as GABA and endogenous opioids not being released, causing a heightened response to the stimulus. |
Allodynia (painful response to a stimulus that is not normally painful) | Inhibition of excitatory interneurons between Aβ fibres and fibres detecting noxious stimuli (Aδ and C fibres) by silencing the pre-existing pathway connections so that non-painful stimuli do not cause a painful response. | Inhibition does not occur correctly; there is crosstalk between Aβ fibres and fibres detecting noxious stimuli allowing the spread of excitation from Aβ to Aδ and C fibres, leading to a response of pain to a non-painful stimulus. |
Spontaneous pain (pain in the absence of any stimulus) | Inhibition of neurons in the spinal cord by inhibitory interneurons releasing GABA to prevent spontaneous action potentials leading to a pain signal when there is no stimulus detected. | Inhibition does not occur correctly; not enough or no GABA is released, causing spontaneous pain. |
Radiating pain (pain that radiates out from the stimulus to areas that did not experience the stimulus) | Inhibition of excitatory interneurons that span out to different areas of the body. | Inhibition does not occur, thought to be due to insufficient GABA release. |
Chronic pain follows complicated and in part poorly understood mechanisms. Such long-term pain syndromes are hardly predictable and may be very hard to manage satisfactorily.
Facial arthromyalgia is probably the commonest form of chronic facial pain syndromes seen clinically. This is a combination of myofascial pain from inappropriate stimulus of muscle causing painful spasm, neuropathic response, and is often associated with affect changes suggesting changes in higher cognitive processing of the chronic painful stimuli.
Pain, in particular chronic pain, is an all-encompassing sensation. It can affect the whole body, making it difficult or impossible to go about daily life. It can also be hard to explain to others who have not experienced it why it has such an effect on life, potentially making it a very isolating experience as well. In particular chronic pain affects different people in different ways.
There is no objective or simple way of classifying or ‘measuring’ pain (acute or chronic pain, for that matter). There are a widely used subjective systems of describing how bad the pain is by using numerical systems with a scale of 1 to 10 (or similar), or other variants of a visual analogue scale, or a scale of smiling to crying faces for children. However, such attempts really cannot get around the problem that pain cannot be measured in such simplistic ways. The problem can be communicated to a certain extent with the help of language (including body language) and that also helps to identify the quality of pain sensation rather than just its intensity.