Inflammation
With acute and/or chronic inflammation being the most noticeable sign of the functions and responses of the immune system, we take a closer look at the underlying working mechanisms of the body’s defence system. The most common way to classify the different mechanisms and components at work in the immune system is to subdivide it into a fast-response, non-specific subsystem (the innate immune system; response within minutes or a few hours) and a more sophisticated and specific, slow-response subsystem (the adaptive immune system; response within several days).
These two categories are not completely separate from each other, and both are closely related to the various transport functions of the vascular (blood vessels) system, including many aspects of blood clotting. The vascular system is involved because it is necessary to transport specific molecules and cells to the location in the body where infection and/or other tissue damage requires an inflammatory response. That is, the attempt of the body to eliminate the cause(s) of a problem leading to inflammation.
We use the immune response to bacterial infection as our worked example (see below). However, we emphasise that other causes (biological, chemical and physical) lead to similar types and cascades of immune system responses. In particular, the role of inflammation in wound healing is relevant in the context of maxillofacial surgery (see below). Our worked example indirectly also illustrates, broadly, the many opportunities for small causes and errors leading to big malfunctions in the complicated immune system.
Below we can only provide a rough sketch of how the immune system works (if everything functions as it should) but hopefully this will also give an impression of how complicated in reality this defence system is. Given the importance of the immune system, there is ample literature about the topic, as well as much ongoing research into it, including its failures and options to treat malfunctions of the immune system. The organisation of the human immune system even inspired the construction of intelligent, rule-based machine-learning computer algorithms (alongside many other biologically inspired algorithms).
The innate immune system
Invading pathogens (bacteria, fungi and viruses) typically carry some surface molecules (sugars and proteins) as part of the normal make-up of their surface structure. The precise nature of these surface molecules varies slightly between pathogens but there are common patterns and types of surface molecules (peptidoglycan is an example from the make-up of bacterial cell walls). The innate immune system is organised such that it can rapidly identify the presence of such typical surface molecules.
This makes the innate immune system a general, non-specific rapid response defence against all kinds of invading microorganisms (see Figure 1). The innate immune response makes use of the presence of these common surface molecules by acting as a general sensor, which triggers the mechanisms by which the destruction of the invading species is then attempted.
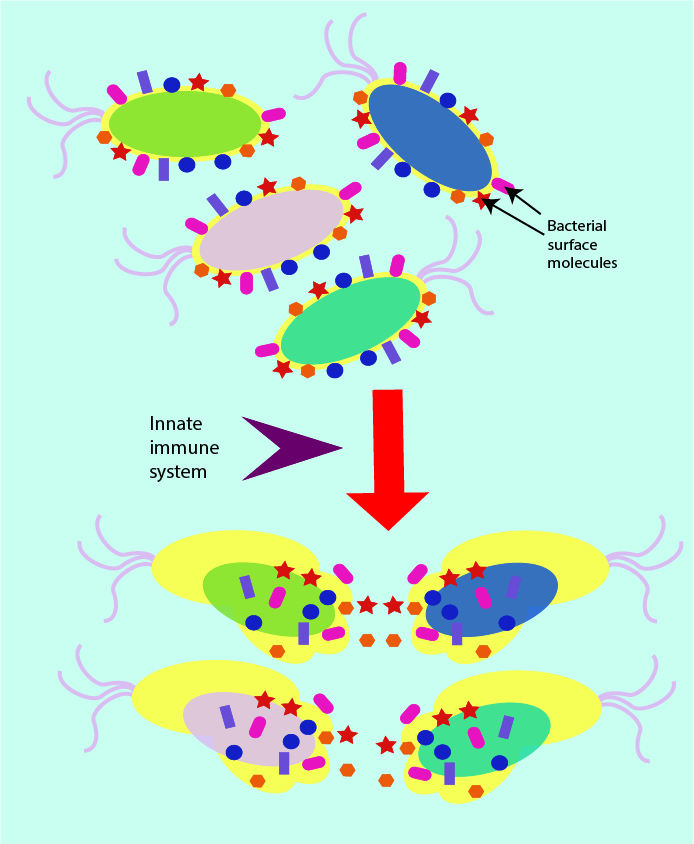
Phagocytes are a type of white blood cells involved in the innate immune response (see Figure 2). Phagocytes can recognise the surface molecules of pathogens through their own surface molecules, called cell surface receptors.
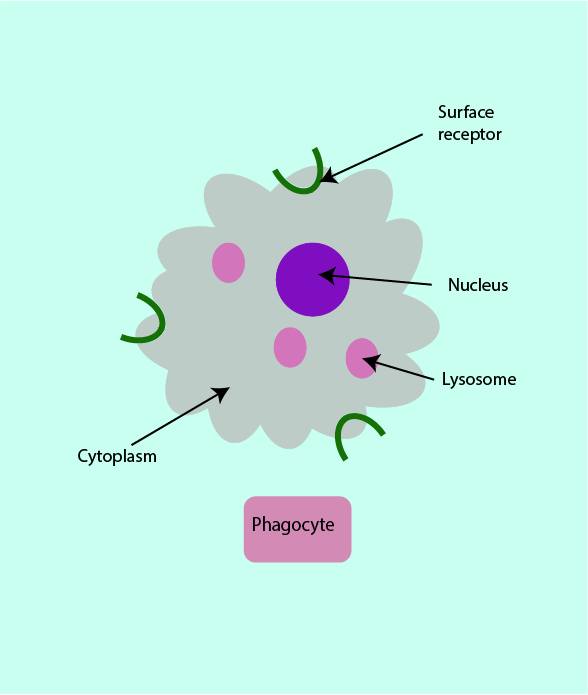
Phagocytes can distinguish between pathogenic cells and human host cells due to some differences in the molecules present on the surface of human cells. On host cells there are often no surface molecules which are complementary (able to fit together) to the phagocytic cell surface receptors. Sometimes surface molecules present on a pathogen may be only slightly, but significantly, different from those on a human host cell. This is schematically illustrated in Figure 3. This distinction is vital so that host cells (often called self-cells) do not stimulate an erroneous immune response, targeting the host. Similarly, it is important that under normal circumstances an immune response is not triggered by the presence of the host’s commensal (friendly) microorganisms.
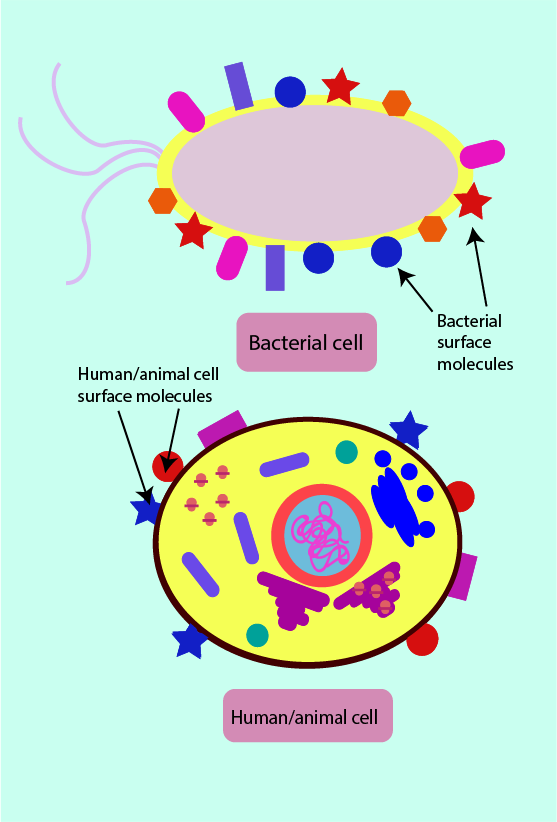
When a phagocyte surface receptor binds to a surface molecule of an invading microorganism, the phagocyte engulfs the pathogen completely by surrounding it in what can be thought of as a bubble (a lysosome; see Figure 4). This process is called phagocytosis. Once the pathogen is encapsulated by the lysosome, the phagocyte produces toxic chemicals inside the lysosome (hydrogen peroxide, superoxide and nitric oxide) in order to destroy the pathogen trapped inside. The chemically decomposed pathogen debris becomes the major component of pus in infected wounds or in an abscess.
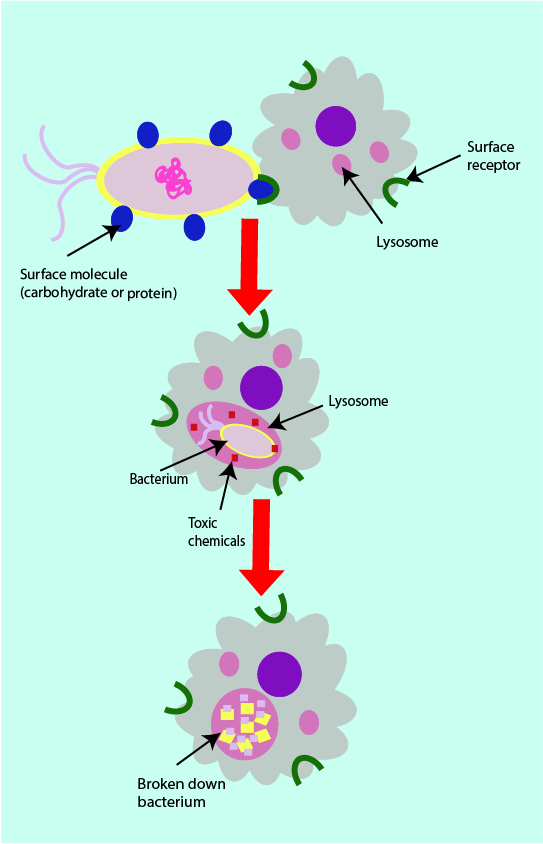
Other receptors on the surface of phagocytes can bind to a range of further molecules on the surface of pathogens and generate what is known as a signal cascade. A set of biochemical signals and reactions, one after another, is triggered which eventually leads to the synthesis of particular proteins in the cell. Such a cascade is schematically illustrated in Figure 5. It is a series of events which involve the DNA contained within the nucleus of a cell (shown in purple). These processes allow for certain genes to be switched on or off, by the means of a protein molecule switch known as a transcription factor. If a gene is switched on, the ‘recipe’ for making the corresponding protein is made available and so the protein can be produced by the cell. A wide range of proteins produced by such signal cascades are involved in the immune response.
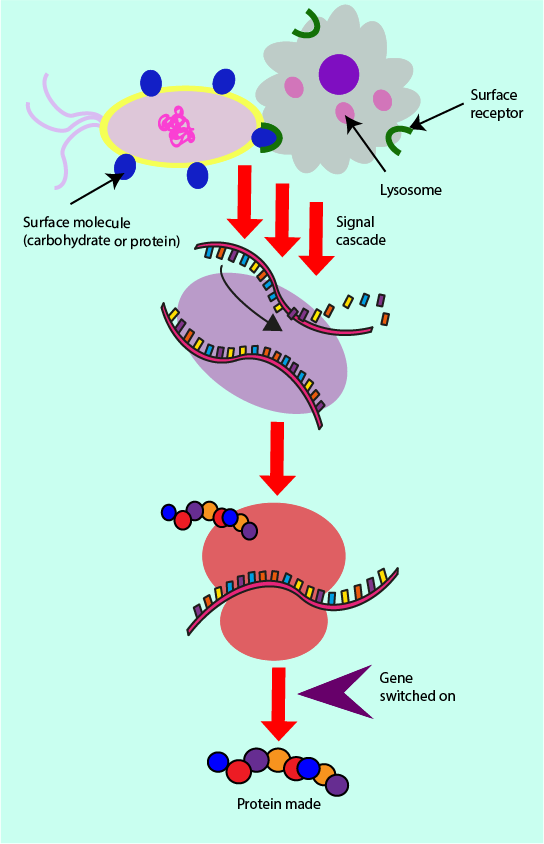
Receptors on the phagocyte surface are specialised protein molecules which are able to bind to different microbial molecules (from bacteria, viruses, fungi) in order to switch on or off gene expression, and hence protein production. Binding to these receptors leads to production of specific signalling proteins, cytokines including chemokines. Such signalling proteins are vital messenger and mediator molecules for the swift execution of the inflammatory response by the innate immune system.
When phagocytes are signalled to produce chemokines through surface receptor binding, release of these chemokines from the phagocytes leads to a chemokine concentration gradient (see Figure 6).
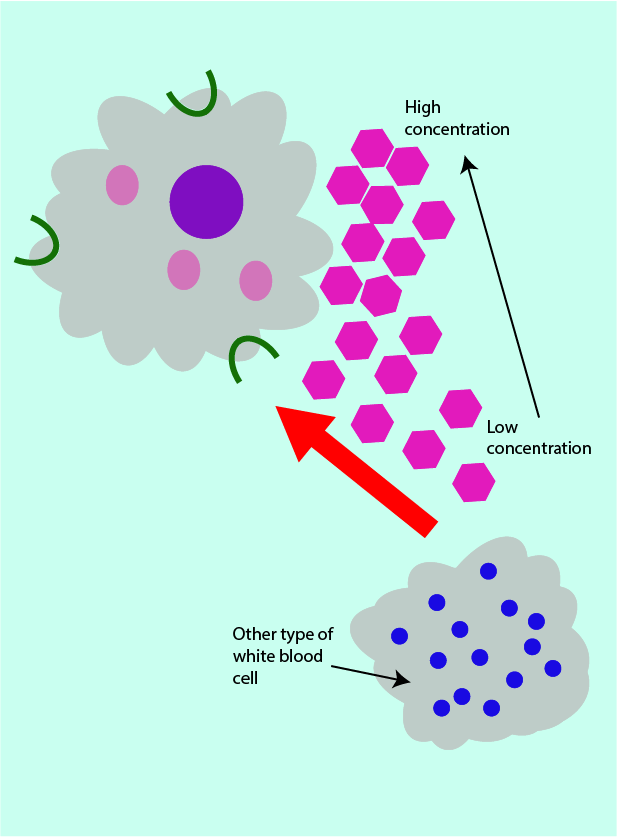
The human body generally makes use of concentration gradients in order to transport and direct a range of materials and cells around the body. In an inflammatory response, the chemokine concentration gradient attracts other types of white blood cells (which can aid the phagocytes in pathogen destruction) to the site of infection.
Phagocytes can produce further types of messenger proteins, such as cytokines that make it easier for some helpful white blood cells to pass from the blood in capillaries (small blood vessels) into infected tissue via endothelial cells (cells which line the capillaries). This type of cytokine achieves this effect by increasing the gaps between endothelial cells (vasodilation; see Figure 7 for a schematic illustration). The movement of the white blood cells from the capillaries into surrounding tissues is termed extravasation.
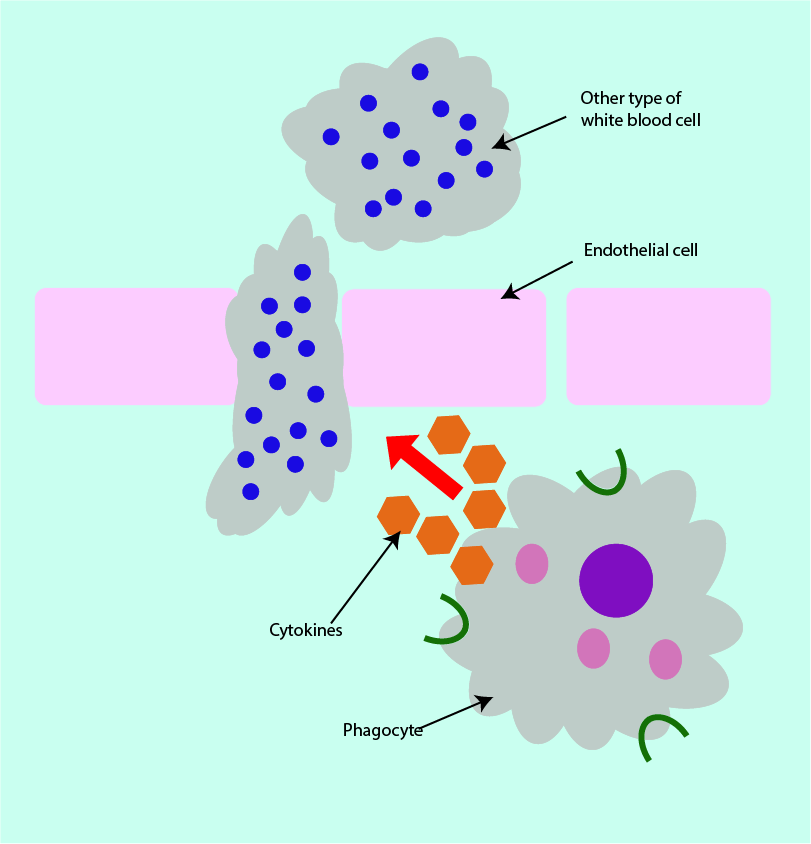
This overall chain of events explains the well-known signs and symptoms of acute inflammation. Vasodilation causes warmth and redness (calor and rubor) of the affected area, extravasation leads to swelling (oedema), pain (dolor) is caused by some of the chemical messenger molecules stimulating nerve endings, and some chemical messenger molecules may cause fever.
The adaptive immune system
The adaptive immune system does not focus on the broad and rapid identification of the presence of pathogens in general, but rather it is able to recognise (and remember) a very large number of molecules, representative of a particular pathogen. This specific recognition is biochemically processed in order to produce an immune response specific to the particular pathogen. It is important that the adaptive immune response is as efficient as possible, it is the last line of defence available to the body against infection. Figure 8 symbolises the specific actions of the adaptive immune system (compare Figure 1, symbolising the general actions of the innate immune system).
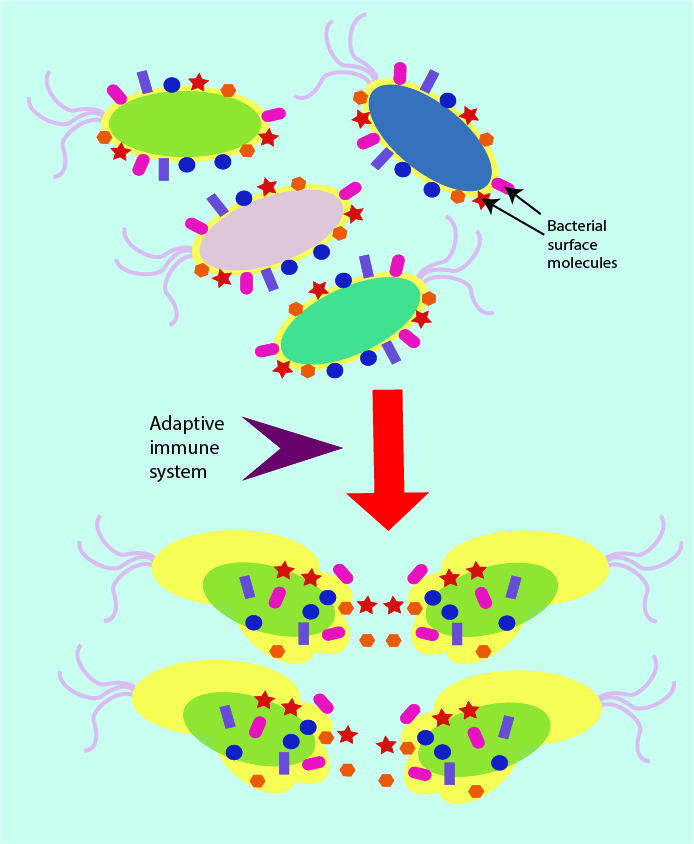
The white blood cells of the adaptive immune system are able to engulf an infecting pathogen, and then identify the various components and molecules which make up this particular microorganism, before they finally present a small protein (an antigen) with a specific shape on their cell surface. In effect, the antigen on the surface of the white blood cell represents, like a fingerprint, the particular pathogen. This type of white blood cell is often referred to as an antigen-presenting cell (see Figure 9).
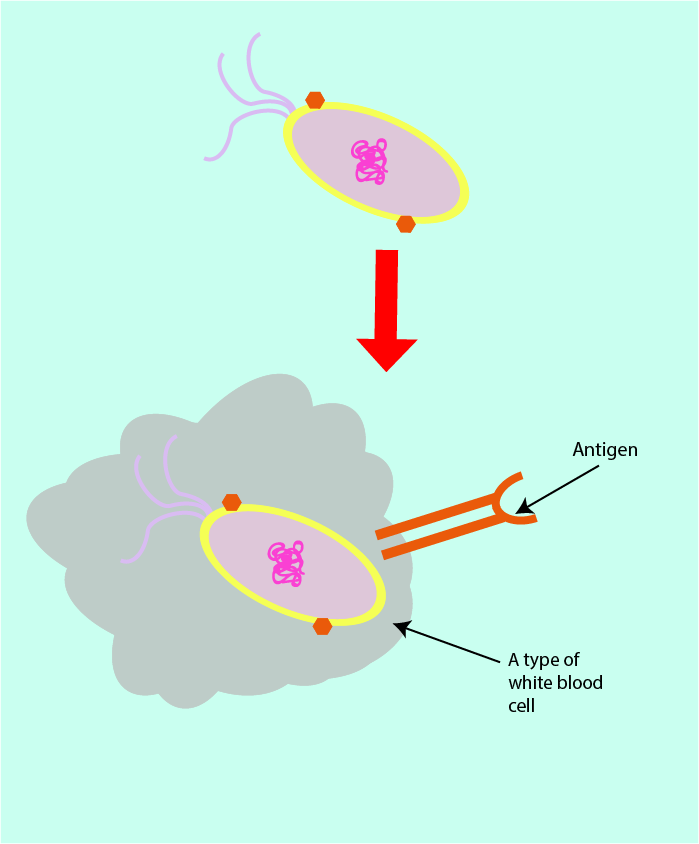
The shape of the antigen on the surface of the white blood cell is complementary (fits exactly) to the shape of the receptor on another type of adaptive immune system defence white blood cell, called a T cell. Binding of the T cell receptor with the antigen signals two separate strands of immune system response (see Figure 11, below).
The first of these responses is called a humoral response. A humoral response is an immune system activity involving extracellular fluids (body fluids) and complements the intracellular immune responses. B cells (another subtype of white blood cells) produce specific molecules, called antibodies. These are proteins which are very specifically matched to the shape of the corresponding antigen. Antibodies have constant regions which are very similar in all antibodies and variable regions which are different for different antibodies and allow specific binding to ‘their’ matched antigens (see Figure 10). This mechanism is often referred to as antibody-mediated immune response.
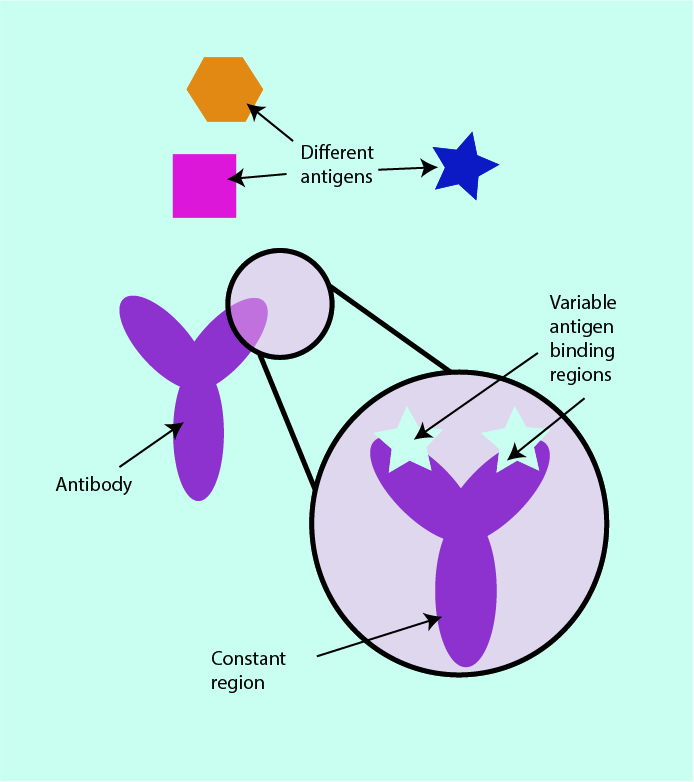
Antibodies have various functions, all reliant upon complementary binding of the corresponding antigen. In this way, antibodies are able to block toxins produced by pathogens from binding to healthy host cells and thus can prevent damage to healthy tissues. Antibodies are also able to form complexes with surface proteins of viruses such that a virus is unable to attach to the host cells and so cannot infect them (this principle is also the mechanism underpinning vaccinations). In similar ways, antibodies can bind to bacterial surface antigens and can prevent bacteria from forming colonies, which makes them individually more vulnerable to destruction and elimination, either by the immune system or by antibacterial agents.
The second of the two strands of adaptive immune system response is mediated by T cells (subtype of white blood cells, also active in the innate immune system). It is a cellular response in which the binding of the antigen with the T cell receptor results in the production of specialised killer cells. These specialised cells are capable of destroying the invading pathogen. They do so by causing holes in the cell membranes of the microorganisms, leading to leakage from the pathogen cells and eventual cell death.
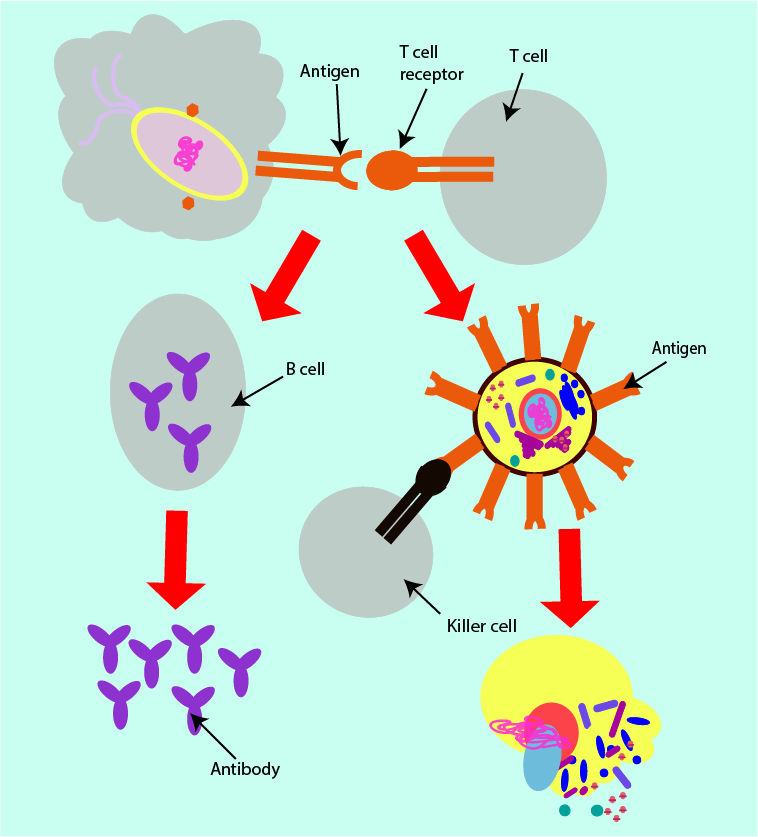
Inflammation and wound healing
Any injury to tissue, from trauma or following surgery triggers an immediate reaction to limit the damage, followed by an orderly and lengthy process of healing. Inflammatory response plays a crucial role in the healing of wounds.
The initial phase immediately after an injury occurs is haemostasis, the attempt to stop blood loss, by way of constriction of injured blood vessels and platelets starting the formation of a sticky fibrin-rich clot. This emergency response occurs immediately, over a few minutes. In addition, platelets release messenger molecules that trigger the start of a rapid inflammatory response. At this stage, vasodilation sets in to ease delivery of helpful cells to the injured tissue site, over a period of about an hour.
Essentially, this very first step toward wound healing involves the build-up of gradients of specific proteins (chemokines and cytokines, see Figure 6; above) that guide immune cells and phagocytes to the site of injury. This phase is the body’s attempt at cleaning a wound before proceeding further with repairing the injured tissue. This stage of acute inflammation lasts for a few days, various mechanisms of inflammatory response orchestrate and guide the process.
The inflammatory response remains active for a couple of weeks, in changing and adapting roles in the progressing healing process. Inflammation is gradually taken over by a phase called proliferation. The initial fast response turns to repair of the tissue damage, still initiated by inflammatory mediators. It is important (from an evolutionary point of view) that initial repair re-establishes functionality to the body after injury relatively quickly. Nature has opted for a staged, two-stage approach to this challenge.
In the proliferation phase, over a period of several weeks to several months, the inflammatory response directs the growth of new blood vessels (angiogenesis) in the injured area. These new blood vessels are needed to transport fibroblasts (cells that produce connective tissue such as collagen and other structural materials in tissue) and macrophages (a specialised type of phagocyte, white blood cell) to the location in need of repair, and to remove debris (diseased tissue, spent repair materials) away from the site. A typically very visible sign of the body’s wound repair at work over this phase is the reddening of the wound, caused by angiogenesis (ingrowth of new blood vessels) and leading to an intermediate period in the healing process where a wound looks raw and seemingly as if it is getting worse rather than better. Over this phase mostly granulation tissue is formed, new connective tissue with numerous microscopic blood vessels. Granulation tissue grows from the inside of a wound and eventually fills the entire damaged space while some contraction of the damaged site occurs as well. This initial repair stage delivers a degree of functionality and wound closure, about 50 percent, or so, of the original tissue strength and removes the urgency of the body response.
Gradually, the proliferation phase then moves on to the last phase of the wound healing, the maturation and remodelling phase. This final stage takes up to two years for completion and is essentially an optimisation of the initial repair results from the proliferation phase. It makes sense that this last optimisation phase is slow: at this stage the focus is moving to an optimal long-term result, and that is best delivered in a slow and non-chaotic process of replacing the initially deposited ‘quick fix’ connective tissue material by better quality connective tissue (there are different types of collagen, for example). The inflammatory response is now much reduced, the structure and strength of the local tissue is gradually improved. A body is a highly efficient system, nothing goes to waste and nothing unnecessary will be preserved. Accordingly, over the maturation process the multitude of local new blood vessels around a site of injury and scar are gradually reduced, in line with the reduced need for transport to / from the injury location. The visible sign of this process is the corresponding gradual change in colour of a maturing scar, which over time turns pale and becomes much less visible.
A typical result of wound remodelling delivers roughly 80 percent of the original tissue strength. The ‘auto-repair’ process of wound healing is thus a slightly incomplete and imperfect type of tissue regeneration when, for example, compared with the normal renewal processes of intact skin where the regeneration is complete and perfect.
It is sometimes said that scars resulting from the wound healing process are the price for the swift on-set of the healing process after injury. There are some noticeable exceptions where no scars are formed in the healing process, for example superficial scratches, tattoos, or the healing of skin wounds in a foetus. Also, some tissues heal with reduced levels of scarring, for example the oral mucosa (lining of the mouth). Oddly, the latter two examples have in common a substantially reduced inflammatory response early in the healing process. These differences in wound healing mechanisms are the subject of much ongoing research with a view to improve care and outcomes for problematic wounds.
A number of problems in the wound healing process can occur for people with underlying conditions that modify or impair the immune response.
Lack of formation of scar tissue leads to rupture of wounds (dehiscence). Another well know example of problematic wound healing is the development of chronic non-healing wounds in people with diabetes where an aberrant immune response prevents, or at least considerably reduces, wound healing. Such chronic wounds and ulcers not only increase the risk of infections and necrosis but typically also have negative effects on quality of life.
An example of malfunctioning wound healing, likely to result from an overactive inflammatory response to injury, is the formation of keloids. Keloids are essentially excessive scar tissue, continuously formed beyond the original area of injury. Keloids mostly affect dark-skinned people. Hypertrophic scarring is another form of excess formation of scar tissue but is localised to the original area of injury. Aberrant orchestration of healing events in the proliferation phase may lead to excessive formation of granulation tissue. An example of the effects of timing errors in the early inflammatory response is the occurrence of a painful ‘dry socket’ after dental extractions when the initial protecting clot is resolved too rapidly. Combinations of slight orchestration misalignments of the healing process with occasional mechanical problems may lead to deficient contraction (affecting skin grafts) or to excessive contraction (a typical long-term problem arising from large burns). Another potential problem (mostly affecting burn wounds) are changes in pigmentation of scar tissue.