Physical principles (Radiotherapy)
Contents
Trying to sketch the basic working principles of radiotherapy is a multi-faceted task that is not really possible to achieve by simply reducing it to an isolated view of the physics of beams of particles or photons. A great deal is known about these basic physical principles but here we need to also make connections to the ways in which radiation interacts with matter, in particular the effects of high-energy radiation on living matter. An enormous body of scientific literature about this topic exists and basic research as well as clinical trials are underway in many places and laboratories.
Our brief discussion takes a look at the energy range over which photon radiation occurs and puts high-energy radiation into context with the energetics of molecules in cells. The different ways in which high-energy radiation causes profound biological damage are outlined, depending on the type of radiation used (photons or particles). Strategies to make photon irradiation regimens more selective and less damaging to healthy tissue are briefly mentioned.
The range of electromagnetic radiation and its high-energy region
Radiation by photons covers an extremely large range of frequencies and, accordingly, energies. This is summarized in Figure 1.
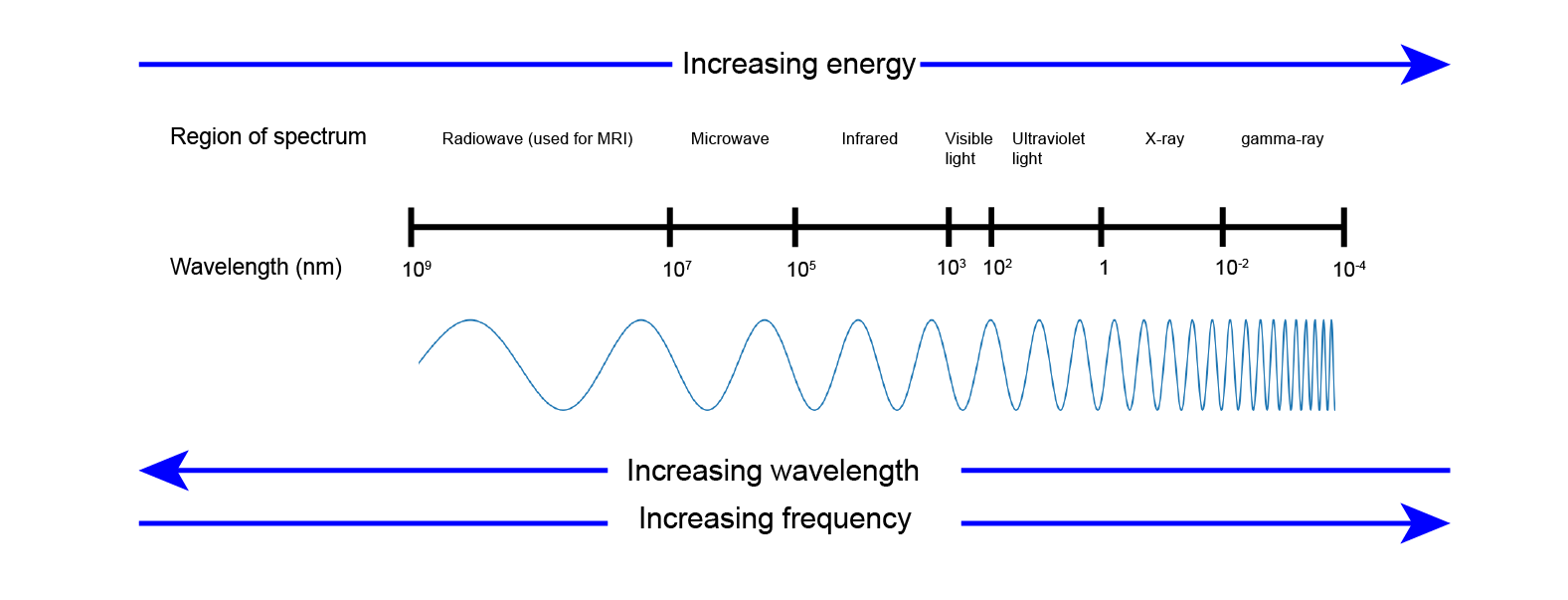
This overview of the range of photon radiation with the approximate frequencies / energy ranges associated with the different regions is known as the spectrum of electromagnetic radiation. Roughly the region of UV (ultraviolet) radiation marks the energy that is high enough to damage the organic molecules that make up living cells and bodies. Chemical bonds holding together such molecules have typical strengths (measured in kJ/mol) for different types of bonds, about 400-500 kJ/mol for a standard covalent bond in standard organic molecules. Most of the organic molecules that make up the cells in our bodies belong to the type of standard organic molecules.
In order to damage and break a chemical bond in a molecule by radiation the energy of the radiation has to be at least of the same order as the strength of the chemical bond. All the types of radiation to the left of the UV region in Figure 1 don’t carry enough energy to be able to damage or break chemical bonds in typical molecules. The energy of ultrasound or radio waves is many orders of magnitude less than the strength of any chemical bond and thus ultrasound or MRI scans are not harmful.
UV radiation is the borderline energy region. The higher-energy end of the UV region of radiation undoubtedly does damage to living cells (think of damage to skin by sunburn and the occurrence of skin cancers due to unprotected and extended exposure to intense sun light, or tanning studios) and it makes good sense to protect skin and eyes from these effects. The lower-energy end of the UV region can still be harmful as accumulated effects occur over time. For example, repeated sunburns or the ageing effects of tanning on skin (wrinkles caused by accumulated damage to collagen molecules in the tissue underneath skin) fall into this category; plant leaves that usually work in photosynthesis can suffer sunburn effects similar to sunburn of the skin of humans. The effects of (UV) irradiation can accumulate over time with the overall damaging effects being dependent on the dosage, that is on overall energy delivered over time.
To the right of the UV region in Figure 1 are X-ray and gamma ray photons, with gamma rays typically being the product of a range of different radioactive decay mechanisms. The energies of X-rays and gamma rays greatly exceed the strength of typical chemical bonds. Thus photon radiation over the entire X-ray and gamma ray energy region lends itself to be used as a destructive force, for example when trying to destroy malignant cells but invariably will also damage healthy tissues. Calling the higher-energy region gamma-rays and the lower-energy region X-rays in this range is largely for historical reasons (when in the past only limited technologies existed to produce high-energy photons and only radioactive decay existed as a source of very high-energy photons).
Gamma rays occur naturally in our environment as the product of radioactive decay of certain elements and/or isotopes of elements, or can be generated in accelerators. The enormous energies released in the process of radioactive decay demand extensive measures of safety and protection to be put in place to avoid catastrophic effects from accidental exposure (think of nuclear power plants). Elsewhere in the universe high-energy processes are ongoing that produce photon radiation in the entire X-ray region of the spectrum (for example, cosmic rays originating from the explosion of supernovae). In our natural environment on earth - luckily - no such processes occur naturally and photons in the X-ray range of energy have to be generated artificially. Creating X-rays basically requires a vacuum tube, fitted with a heated cathode from which electrons escape. A high voltage applied across the vacuum tube accelerates these electrons. The anode in an X-ray tube is a metal plate. When the accelerated electrons hit this metal target, high-energy photons are released – the X-rays. This process can be tuned to produce X-rays over a fair range of energies (and in fairly straightforward ways in modern linear accelerators). For example, X-ray radiation for diagnostic purposes uses the lowest possible energy and dose of irradiation in order to keep potential harm at a minimum.
Biological damage caused by high-energy photon radiation
High-energy radiation mostly harms cells by damaging the DNA molecules in some way. This does not necessarily mean immediate apoptosis (cell death) but functioning DNA is central to successful cell replication and tissue growth. Unrepaired damage to DNA hinders cell replication and eventually prevents further cell growth. Having said that the harmful potential of high-energy photons relies on their energy greatly exceeding that of typical chemical bonds in organic molecules (these chemical bonds are made up by pairs of tightly connected electrons), one might be tempted to assume that the direct process shown in Figure 2 is the main mechanism to damage DNA molecules: a direct effect, simply breaking chemical bonds in the DNA strands.
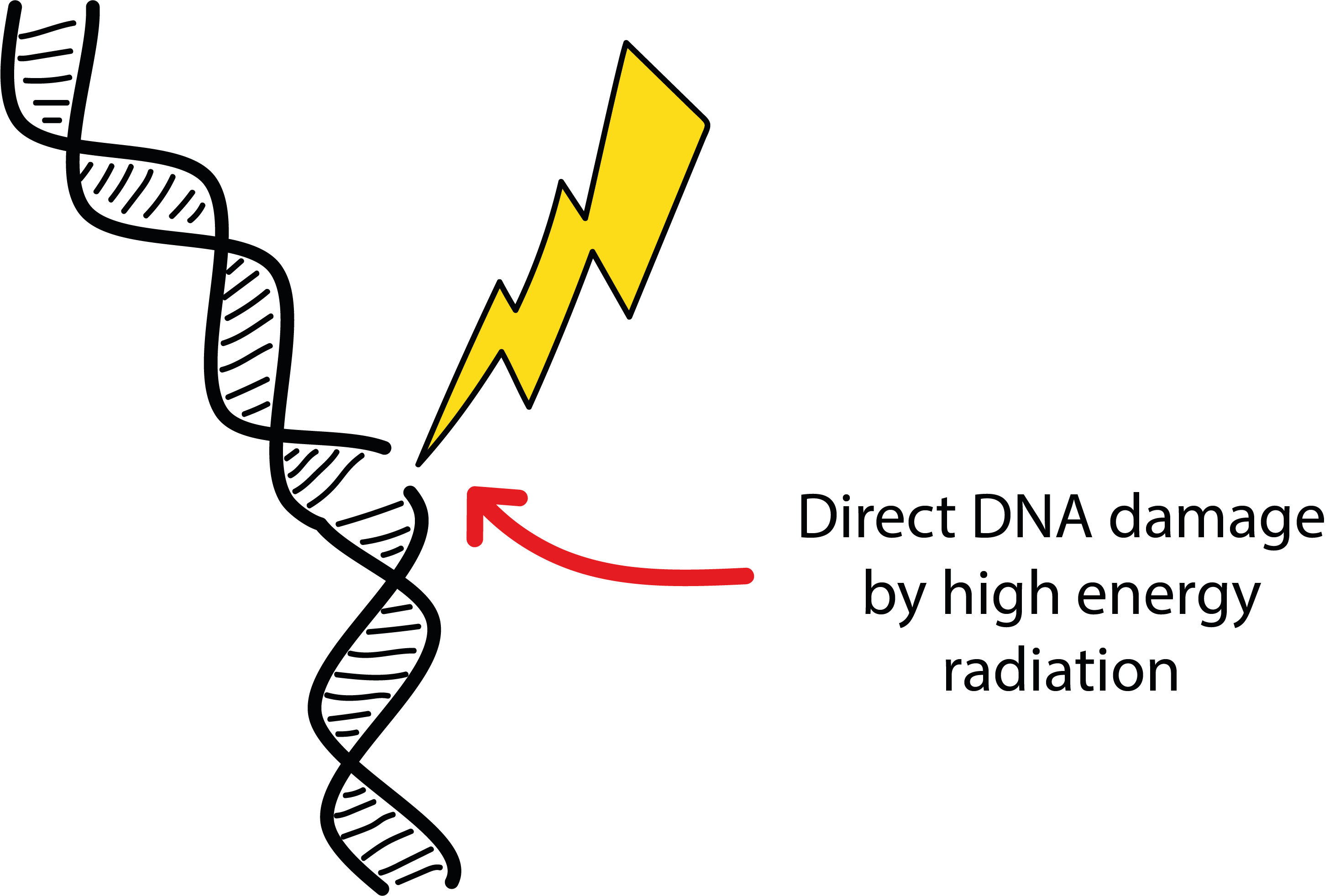
This effect does occur but is not the most potent mechanism by which high-energy photons harm DNA in cells. The main damage caused by high-energy photons is due to more indirect mechanisms. This is illustrated in Figure 3.
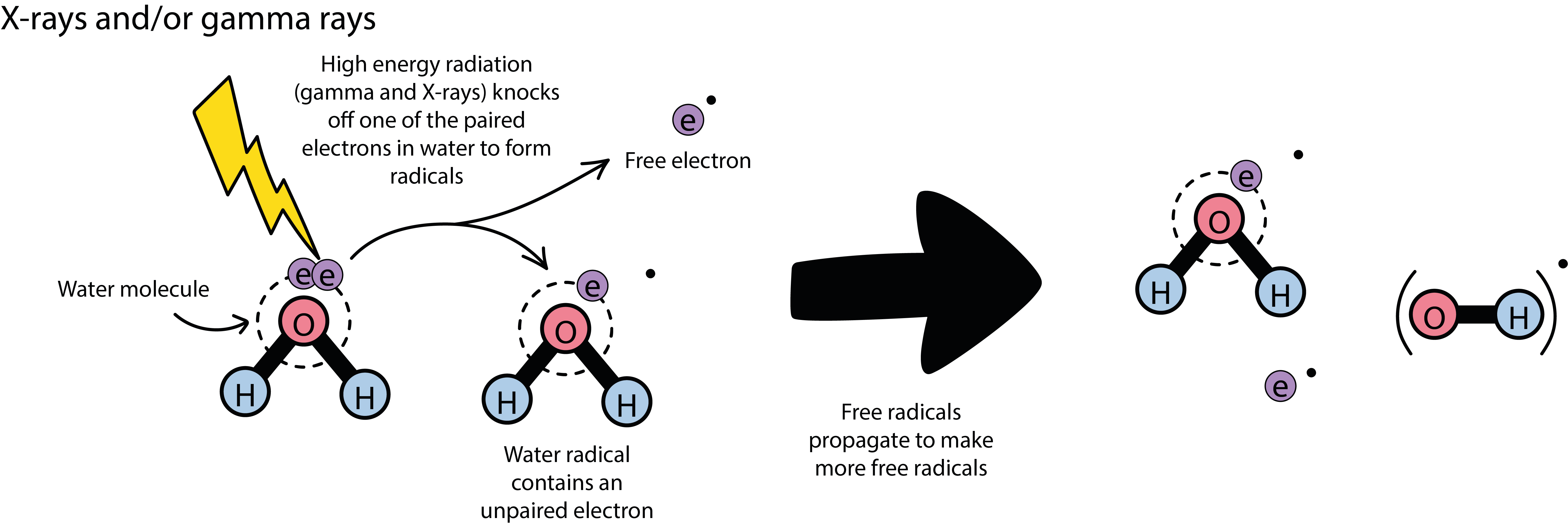
Water, H2O, and oxygen, O2, molecules are basic components in all living cells, water molecules are ubiquitous. Both these small molecules have exposed pairs of electrons that are particularly prone to interact with high-energy photons. High-energy photons simply knock out an electron, creating a so called free radical in the process (a radical is a molecule with an unpaired electron). Radicals are highly reactive chemical species, especially those created from O2 molecules, mostly OH• radicals. It is these radicals that cause and mediate damage to the DNA in the cell as well as preventing cellular repair mechanisms. In addition, each radical starts a chain of events of creating further radicals, propagating and multiplying the harmful mechanism beyond the actual duration of irradiation. The different types of indirect damage to DNA are illustrated in Figure 4.
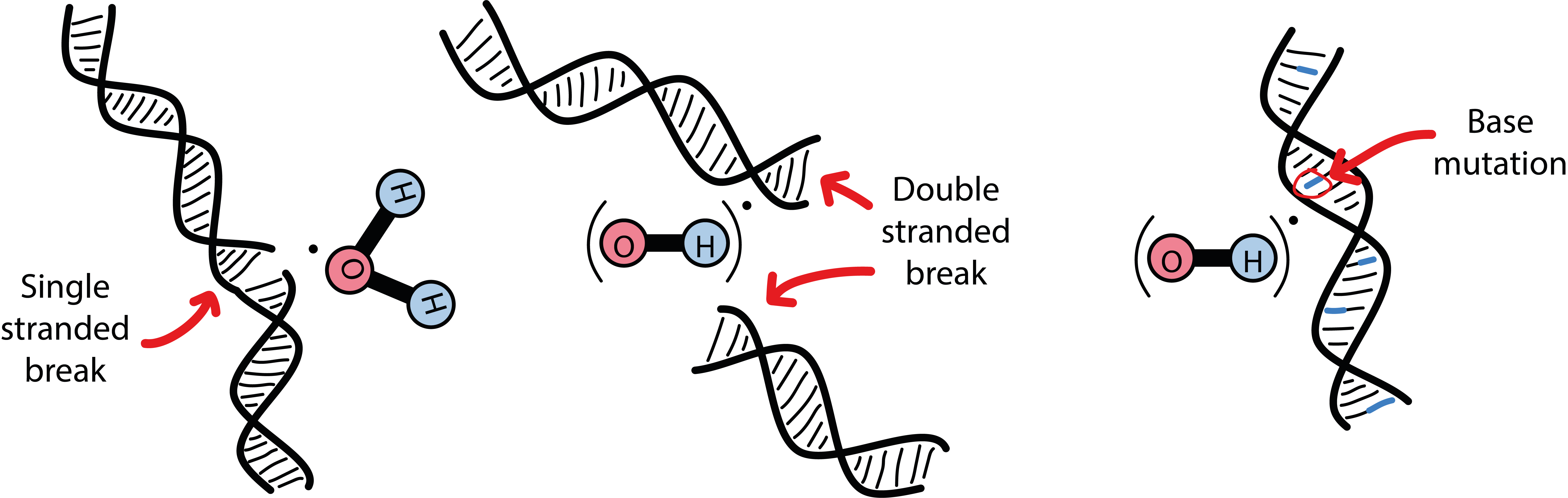
Damage could affect only one of the two strands of a DNA molecule (Figure 4, left), it could cause a double-strand break (Figure 4, middle) or could induce more subtle chemical modifications, so-called base mutations (Figure 4, right). The latter leads to a faulty copy of DNA that will not work properly anymore and will eventually prevent the cell from replicating. Base mutations are thought to be difficult to repair for the cell. It is further thought that double-stranded breaks of DNA are harder to repair for the cell and cause even more severe damage than single-stranded breaks (most of the DNA damage caused by high-energy photons are single-stranded breaks). There are many clever repair mechanisms in place in all living cells, including malignant cells, to fight damage to DNA to protect the life cycle. Malignant cells have such defenses in place in differing degrees of effectiveness, depending on the tumor cell line. Some such cell lines have particularly good defense strategies and hence are generally fairly resistant to radiotherapies (for example, melanoma cells or nerve cell tumours), some other cell lines actually learn how to react to radiation-induced DNA damage and become less vulnerable to radiation over the course of treatment (similar to defense mechanisms in place to protect cells against damage caused by various chemotherapy agents and also leading to resistance).
It is tempting to think that the mechanism by which irradiation with high-energy photons damages DNA is not so important, as long as malignant cells are being damaged. The underlying mechanism, however, is the reason why in some cases radiotherapy does not work as effectively as one would wish, or may require longer and more intense irradiation regimens than one would wish. With most of the cell damage from photon irradiation relying on an indirect mechanism involving various species of oxygen radicals at different stages, some metabolic peculiarities of malignant cells play an important role. Many tumour cells are depleted of oxygen. This could be for the simple reason that the orderly building of cellular energy-supply mechanisms (normally depending heavily on oxygen molecules from regular blood supply) cannot keep pace with the rapid growth of malignant cells, but further, more complicated biochemical and physiological reasons may be equally responsible. Good levels of oxygenation in cells are closely correlated with success of irradiation schemes. Oxygen is an effective so called radio-sensitizer (see below) which is thought to be mainly involved in preventing repair of DNA damage caused by high-energy radiation.
Low levels of oxygen in tumour cells is called tumour hypoxia. Oxygen-depleted tumour cells instead tend to switch to high rates of glucose (sugar) uptake to make increased glycolysis their path to sustain their energy demands (this increased rate of glucose uptake is exploited in PET scans to identify and locate malignant growth in the body) as one of their many survival strategies. Hypoxic cells respond less sensitively to high-energy photon irradiation. Some of many past and current research activities therefore look into possibilities to increase the oxygen levels in hypoxic cells, or to create similar sensitizing effects in some other ways. For example, hyperbaric oxygen has been suggested (and tried). Use of other synthetic radio-sensitizers such as NO• gas has been explored, and some of the common chemotherapy agents seem to have enhancing effects on irradiation schemes (as well as on unwanted side-effects), presumably by hindering DNA repair. Many other biochemically inspired choices of compounds have been, and continue to be, explored for their potential to act as radio-sensitizers. Other ideas involve the switching off of regulatory proteins in cells that mediate and manage the cells’ response to hypoxia by using inhibitors for such protein actions (HIF-1 (hypoxia-inducible factor) inhibitors) and in this way preventing increased uptake of glucose instead of oxygen. The general aim of all such research activities is to selectively enhance the sensitivity of malignant cells to the effects of high-energy radiation. Convincing evidence of the overall beneficial effects of many such strategies in vivo from high-quality trials is yet to be seen.
Generating high-energy radiation with different particles
So far we have only considered high-energy radiation delivered by photons. Photons may be seen as little packages of light waves carrying energy depending on the frequency of the light wave: the higher the frequency of the wave, the higher its energy. Only a very small section in the frequency range of electromagnetic radiation is the area of light waves that are visible for us (see Figure 1).
High-energy radiation can alternatively be delivered by beams of small particles if these are travelling sufficiently fast, at a considerable fraction of the speed of light. Creation of particle beams suitable for therapeutic irradiation thus requires local access to cyclotron or linear accelerator technologies. In principle, many different small particles and ions could be delivery vehicles for irradiation. Of these only a few types of particles are practically useful: electrons, neutrons and protons. The general motivation to explore alternative sources of radiation stems from the need for more specific irradiation schemes, and/or the need for different mechanisms to cause damage to cells from the indirect effects of photon irradiation.
Electrons
High-energy radiation is by far most commonly delivery by photons, produced in small linear accelerators optimized for delivery of photons in the X-ray region for purposes of radiotherapy. X-ray photons are created by accelerated electrons hitting a metal target which then releases the high-energy photons. Many clinical linear accelerators are constructed in such a way that the last step in the process can be omitted and the accelerated electrons can be collimated and used as delivery vehicles for irradiation, instead of producing photons.
Electron irradiation schemes are the second most common schemes. Particle irradiation tends to cause more direct damage to cell structures than does irradiation by photons, potentially causing more severe damage to malignant (and healthy) cells. Beams of accelerated electrons only reach body regions near the surface and thus are only suitable for irradiation of tumours on or very near to the body surface, traditionally superficial skin cancers. This is in part due to the fact that electrons undergo multiple scattering events while travelling along and thus get delineated from a straight trajectory as well as loosing energy rapidly while travelling through matter (Bragg peak, see below and Figure 5). This limited penetration depth may be viewed as a blessing or a curse: limited penetration depth means less damage to healthy tissues deeper in the body but electrons cannot reach target regions deeper in the body.
Electrons carry a negative charge and thus have many different ways to interact with matter / body tissues. Carrying charge is the main reason for their multiple scattering events when travelling in matter. Body matter is quite irregularly structured and made of many different components, and bodies overall have irregular shapes. This presents the main challenge to deliver precise electron irradiation schemes, even if mainly aimed at superficial body regions: the multiple interaction mechanisms of accelerated electrons with matter, combined with the different irregularities of body shape and structure make it challenging to model and pre-calculate precise irradiation schemes for electron irradiation.
Neutrons
The neutron is another particle that can be accelerated (though a major reactor and accelerator facility is required; accelerated neutrons are usually derived from beams of accelerated protons) to speeds that make it suitable for therapeutic high-energy irradiation. As the name implies, neutrons carry some mass but do not carry charge and thus do not suffer the same kind of multiple, charge-related different scattering events as electrons. However, fast neutrons are not widely available, only a few centres worldwide are equipped to deliver suitable neutron beams. The practical requirements to house such a facility (essentially a vault accommodating the equipment and irradiation sessions, acting as a radiation shield to the environment) are also demanding.
It is thought that once hitting their target, neutrons cause severe destruction to cells by directly damaging DNA (lethal double-strand breaks and other direct structural damage, see Figures 2 and 4). In principle, this makes neutron (and other particle) irradiation schemes promising approaches to cause more damage to hypoxic malignant cells than photon irradiation can achieve. While this may be a wanted effect as far as malignant cells are concerned, the undesired effects on healthy tissue are more severe as well. Neutron irradiation is not likely to become a widely used irradiation scheme any time soon, given the enormous logistical difficulties and cost involved and especially as there do not seem to be overall therapeutic advantages over modern photon irradiation schemes. In addition the unwanted effects of so-called fast neutron therapy were so devastating in the initial clinical trials that they were abandoned.
Protons
Yet another particle with properties suitable as a delivery vehicle for high-energy irradiation is the proton, the nucleus of a hydrogen atom carrying some mass and a positive charge. Like irradiation with other particles it is expected that irradiation with proton beams causes more direct damage to DNA and cells than photon irradiation, mostly causing more double-strand breaks.
Protons can be accelerated to particular speeds / particular energies. A particular energy corresponds to a specific penetration depth in matter / in the body where their highest dose of radiation will be deposited. This is different from the way how, and where, the highest dose of photon radiation is deposited. The two mechanisms are compared in Figure 5.
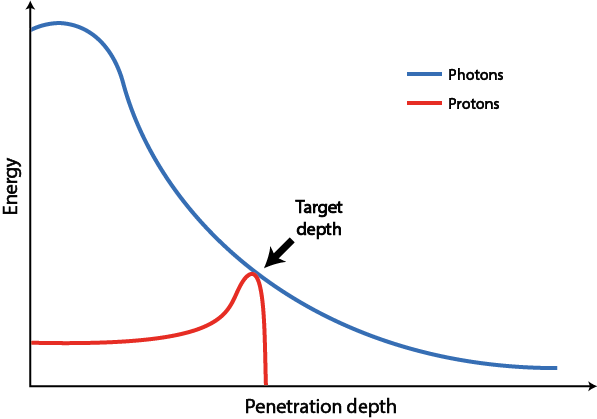
The deposition of radiation of photons essentially decays exponentially with increasing penetration depth, exposing tissues between body surface and target area to high doses of radiation. The deposition characteristics of protons are quite different: as accelerated protons travel through matter, they lose only little energy and by far the largest proportion of radiation dose is deposited in a small area defined by their initial speed / energy (and the material through which they travel). This spot is called a Bragg peak (after its discoverer W. Bragg). Beyond this pre-determined penetration depth there is a sharp drop of energy and hardly any further radiation to tissue within a range of millimeters, in contrast to photons which continue to deliver radiation to tissue beyond the target area.
Protons have mass and a positive charge and, therefore, can be accelerated precisely. Strong external magnets then focus the protons into beams of a few millimeters and multiple beams (‘pencil beam’) are directed by the magnetic fields. Multiple combined and superimposed such beams (several thousand) in principle can then accurately and evenly deliver the required radiation dose to any shape of tumour. Radiation delivery requires reverse planning: first the area and volume in need of irradiation is defined and located (usually by MRI and/or CT scans) and then the best possible irradiation scheme is derived. This is not a straightforward process as planning needs to include a multitude of parameters and interdependencies of parameters – bodies are irregularly shaped and are made up of heterogeneous structures. Typically, suitable irradiation schemes are worked out by numerical optimization and repeated checks are carried out throughout the treatment scheme.
Proton radiation therapies are considerably more expensive than photon radiation schemes and can only be offered in specialised centres with technology to accelerate and focus proton beams. In the UK, proton radiation is offered very selectively. So far, only relatively low-energy protons are regularly used for the treatment of eye tumours in one centre. However, two new proton irradiation facilities are scheduled to be opened in 2018. It is likely that these facilities will be mainly used for the treatment of some paediatric cancers as well as for some specific types of tumours in adults: hard-to-reach tumours (such as at the base of the skull) and tumours in the vicinity of vital structures preventing conventional photon radiotherapy. From currently available data it is not possible to conclude robustly if there are any significantly improved outcomes from proton irradiation, other than reduced damage to healthy tissue.
Selective photon irradiation schemes
High-energy radiation causes severe damage to healthy and malignant cells alike; so any beneficial applications of radiation therapies will have to maximize radiation delivery to target volumes while minimizing exposure of nearby healthy tissues. Accordingly, the development and implementation of selective photon irradiation delivery schemes is a big theme in past and present research and in the application of radiotherapies.
One long established way to deliver relatively localised high-energy photon irradiation is to implant radioactive material directly into or very near to a tumour (brachytherapy), usually iridium wires. This technique employs the 192Ir isotope which is the most stable of several radioactive iridium isotopes (half-life of ca. two months) and emits both beta particles and gamma-ray photons. The exact irradiation energy delivered by radioactive elements is specific to a given isotope.
Given the trajectories of radiation delivery of photons (see Figure 5) it is obvious that any schemes that permit a more selective delivery of photon radiation to target areas, sparing healthy tissue as much as possible, is highly desirable. For photon irradiation schemes in the head and neck region there are mainly three areas that should be exposed to as little high-energy irradiation as possible: i) the mandible, ii) the salivary glands, iii) generally, soft tissue in the region. Bone is dense material of the kind that readily absorbs high-energy photons (and neutrons in particular). The mandible consists of particularly dense bone and is thus particularly vulnerable to suffer radiation damage such as osteoradionecrosis, ORN. Exposure of the salivary glands to high doses of high-energy radiation can temporarily or even permanently seriously damage the salivary glands, leading to severe cases of dry mouth, xerostomia. High-energy radiation injury of soft tissues triggers physiological repair mechanisms with excessive fibrosis, similar to excessive scar tissue formation from burn wounds, leading to sometimes severely restricted mouth opening, trismus. These are serious and lasting side-effects of non-selective high-energy photon irradiation in the head and neck region.
So called intensity-modulated radiation therapy (IMRT) schemes have become routinely available for photon irradiation in most places over the past 10 years. Similar in spirit to the shape-selective delivery of superimposed multiple proton beams (see above), IMRT aims to deliver photon radiation that mimics and traces the shape of a tumour as closely as possible while exposing nearby healthy tissue to lesser radiation doses. The way one can handle and manipulate proton beams is different from the ways photon beams can be manipulated. In order to subdivide the photon beam into multiple smaller beams that then can be combined to achieve volume-selective irradiation, the photon beam is collimated by blocking sections from the beam (by small lead plates). Regimens to combine many different such arrangements into a series of differently modulated photon beams then produces an overall irradiation scheme that can be tailored to mainly hit a predefined shape and volume of a tumour. Similarly to the challenges in proton irradiation schemes, also in IMRT the main challenge remains in the simulation, planning and implementation of sufficiently selective schemes.
More recent research shows that different fractions and timing of irradiation schemes, as well as different energy levels of radiation all have different effects on different cells and DNA. There will definitely not be any simple solutions along the lines of ‘the harder the beam, the better the result’. There is need for much more research in this area where biology and physics meet in order to understand many of these effects in more profound ways. At least for the time being the modelling of selective irradiation schemes in real living bodies remains a hard challenge, more difficult than the actual technical irradiation delivery.