Ultrasound (sonography)
Contents
Ultrasound imaging is a well-established diagnostic modality in a maxillofacial surgery, with a range of applications. Below we give a brief overview of the basic working principles, the most common maxillofacial applications of ultrasound methodology, and a slightly more detailed explanation about the production of sound waves and how these acoustic waves can produce images.
Basic working principles
Ultrasound scans produce images via high frequency sound waves. The frequency range used in this technique (1 to 30 MHz) for medical applications is called ultrasound (‘beyond sound’) because the region is outside the frequency range that we can hear (humans can hear in the range 16 Hz to 20 kHz). Ultrasound scanning relies on a pulse-echo principle, which involves directing an ultrasound wave towards the material being investigated, and then listening for the returning ‘echo’ after the sound has been reflected off the object.
Ultrasound scans are probably best known for their applications in monitoring a foetus throughout pregnancy (‘baby TV’) but are generally widely used for the examination of soft and fluid-filled body parts (such as cysts).
Ultrasound scans only require relatively small and uncomplicated equipment, thus are economic and fairly portable, offering flexibility. This includes use in clinics, at the bedside, or in the operating theatre. Low-power ultrasound is generally used for diagnostic purposes (see below), whereas higher-power versions are used for interventional purposes. Using ultrasound for diagnostic purposes has advantages and disadvantages. For example, the frequency of the ultrasound wave defines the upper limit for the achievable spatial resolution in an ultrasound image, typically a few mm. This resolution is inferior to the resolution of other medical imaging modalities such as MRI or CT scans - but not all investigations benefit from the highest possible spatial resolution of an image.
Ultrasound scans exploit the different speed with which sound waves travel through different kinds of matter. The speed of sound in air (or more generally speaking, gases) is 330 m/s, in water it is 1496 m/s, and in bone it is 3360 m/s. The speed of sound in soft and nearly liquid matter varies slightly, from 1450 m/s to 1600 m/s. This variation is enough to provide some degree of contrast for different soft body tissues such as fat or muscles.
Figure 1 summarises the various effects of matter on the ultrasound wave being emitted by the transducer probe.
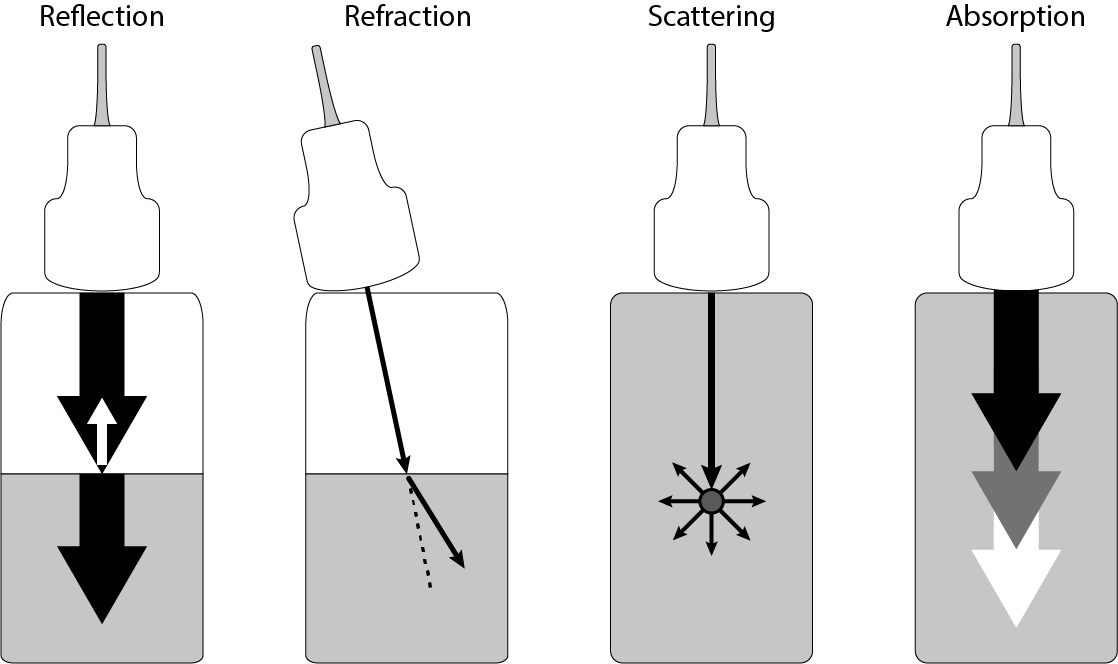
Reflection of a part of the wave at an interface between two tissues with slightly different speed of sound produces an echo in the probe. This is the measured effect that produces the ultrasound image. If the interface is between two materials characterised by vastly different speeds of sound, the wave is completely reflected (for example, between air and skin, or bone and soft tissue) and a ‘shadow’ is created. This is the reason why ultrasound scans cannot ‘see’ beyond bones, and why some gel is applied to the skin before a scan (to avoid air between the skin and the probe).
The other effects in Figure 1 illustrate the losses and the damping the wave experiences while travelling through matter. Some of it is lost due to refraction (where the ultrasound wave changes direction when travelling through the interface (similar to optical waves)). Scattering occurs when the ultrasound wave hits small objects, roughly the size of its wavelength (of the order of 1 to 2 mm), for example small blood vessels. The speckled appearance of ultrasound-scan images is caused by this scattering effect. Finally, some of the ultrasound radiation is absorbed by tissue. The extent to which this happens depends strongly on the type of tissue and to some extent on the exact frequency of the ultrasound used and ultimately can lead to heating of the tissue. However, this is not an issue at the very low power of ultrasound radiation typically used for diagnostic purposes (see Figure 3, below).
Common maxillofacial applications
In a maxillofacial context, ultrasound scans are particularly relevant for investigations of the salivary glands, the thyroid gland, blood vessels and lymph nodes in the neck, other lumps in the neck, the tonsils, and the floor of the mouth. Ultrasound scans work particularly well for the examination of soft tissues close to the body’s surface. More specialised ultrasound scanning techniques can be used to observe blood flow (see below), there are methods to obtain three-dimensional images from ultrasound scans, and ultrasound scanning has been suggested as a diagnostic tool for the examination of some (parts of some) of the cranial nerves (outside the bony confinement of the skull).
The rate of blood flow through arteries and veins can be measured by an ultrasound-scanning technique called Doppler scanning. This technique exploits a phenomenon called the Doppler effect. The Doppler effect is a change in wavelength / frequency of a wave, the source of which is moving relative to the observer (the effect is named after the physicist C. Doppler who first described the phenomenon in the 19th century). The best known everyday-example is the change in pitch heard when a vehicle such as an ambulance is emitting an alarm and approaches, then moves away from an observer (see Figure 2). The pitch heard is higher during the approach and lower as the vehicle moves away.
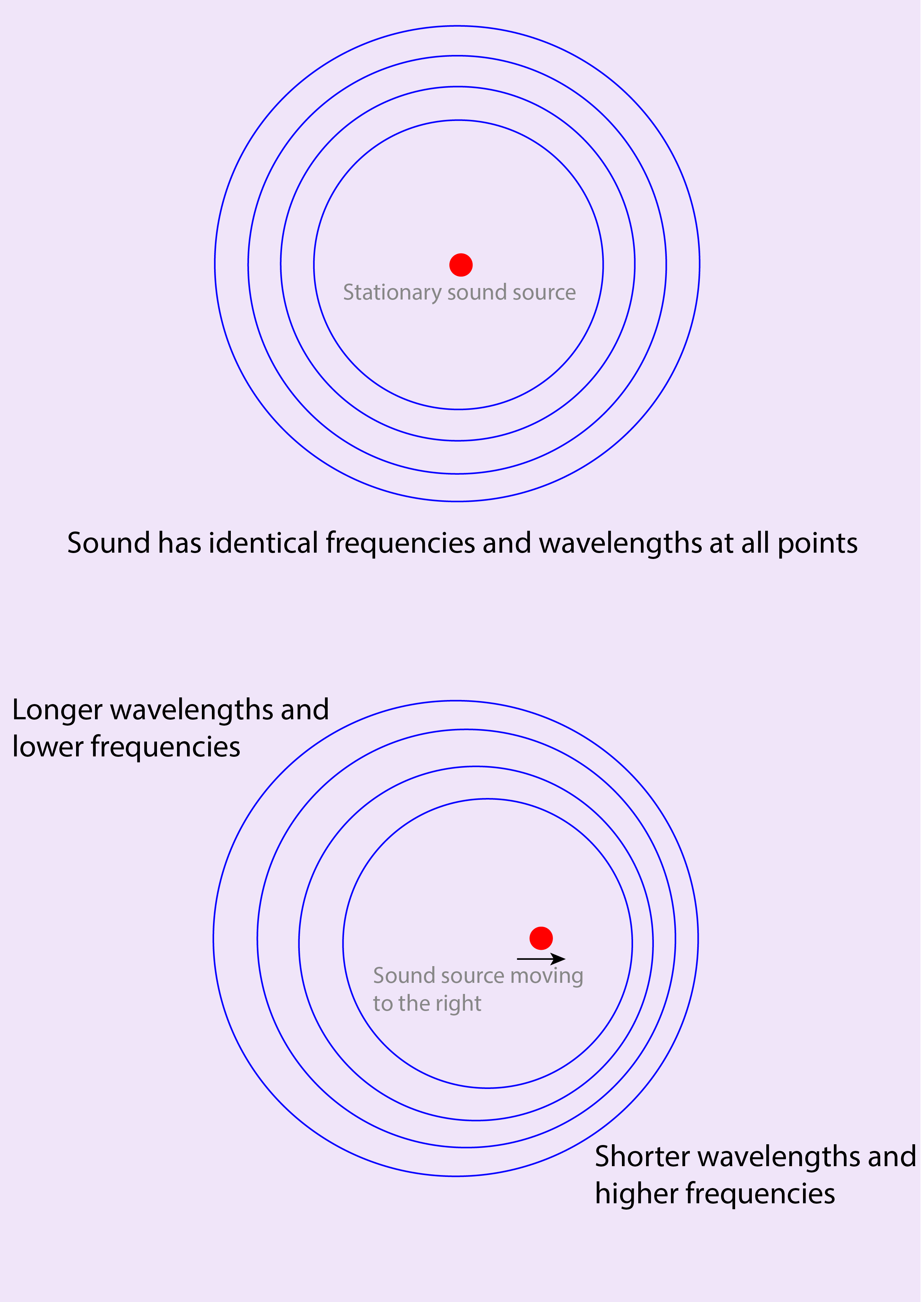
If an object investigated by ultrasound scanning is moving, this will cause the ultrasound echo to be Doppler-shifted to either higher or lower frequencies, depending on its relative motion toward or away from the probe. The magnitude of the shift depends on the velocity of the moving object. Doppler-ultrasound scanning measures the change in echo frequency to determine the velocity of the moving object. An important application of the phenomenon is the examination of blood flow in order to detect blood clots or poor circulation. This is useful, for example, in the monitoring of the blood supply of free flaps after reconstructive surgery (where implantable Doppler probes are used).
In addition, ultrasound scans can provide real-time visual control and guidance for targeted fine-needle biopsies to obtain material for histological examination.
Higher intensity / amplitude of the ultrasound waves can shift their use from diagnostic to therapeutic applications. Some therapeutic applications use short intense blasts of ultrasound directed at the region(s) of interest. In terms of dosage, diagnostic ultrasound applications use intensities of around 0.1 W/cm2, whereas therapeutic ultrasound applications use 1000 W/cm2. An intermediate dosage of ultrasound can be used to provide deep heating to soft tissue within the body. This may be done to increase blood circulation, improve the extensibility of the tissue, or to reduce pain (see Figure 3). The low intensities typically used for diagnostic ultrasound applications do not cause tissue damage. However, local heating effects from medium- to high-power ultrasound applications can cause cell damage.
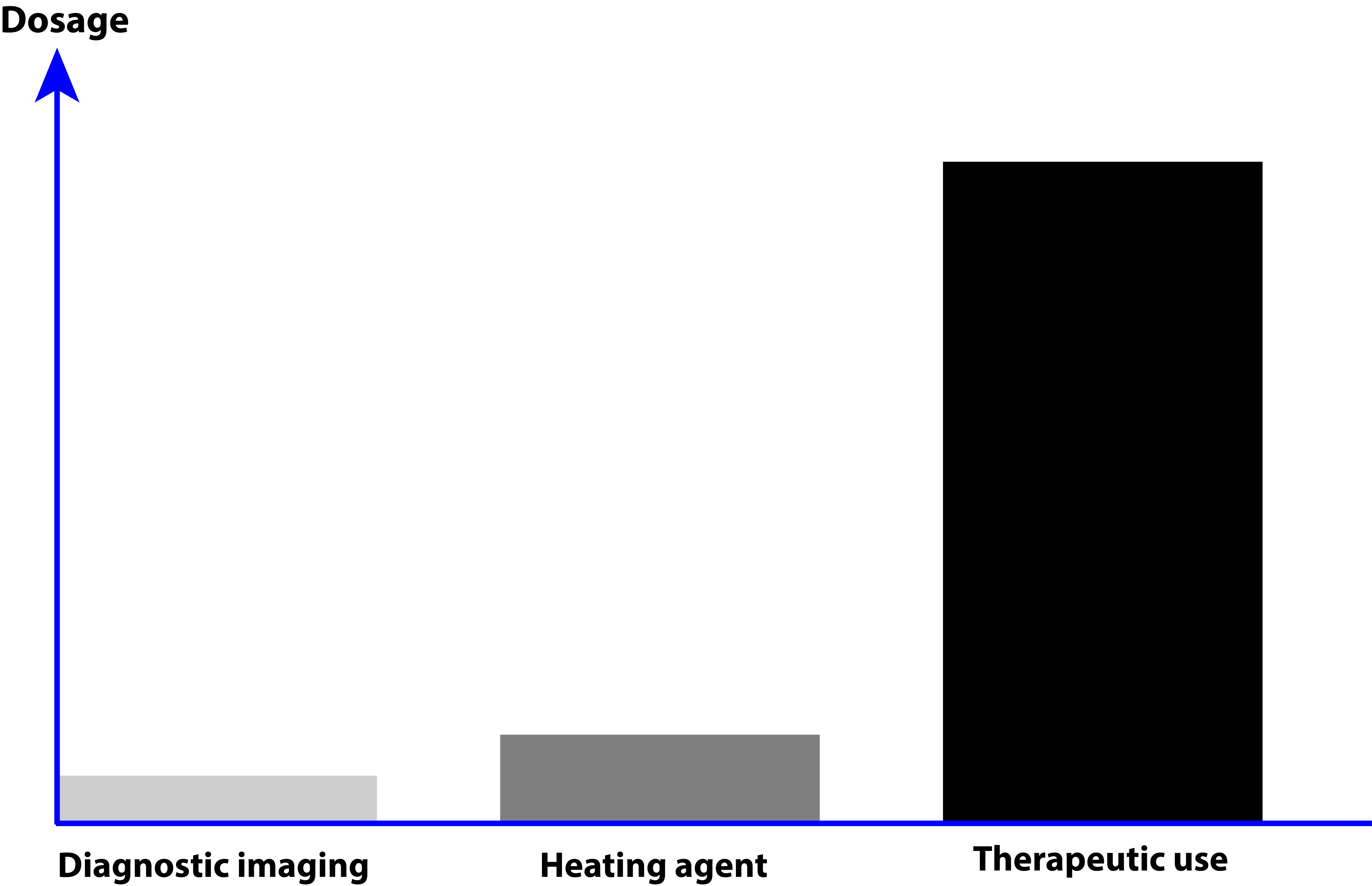
Therapeutic ultrasound applications include the breaking up of calculi (stones) of the salivary glands and blood clots. The ultrasound range 25 to 50 kHz is used for a variety of industrial cleaning purposes, and is also used for the professional removal of dental plaque and particularly calculus. Some endodontic procedures (root canal treatment) with the need to access hard-to-reach spaces benefit from the miniaturisation of the instrumentation: an ultrasonic tip is much smaller than a mechanical handpiece (Figure 4).
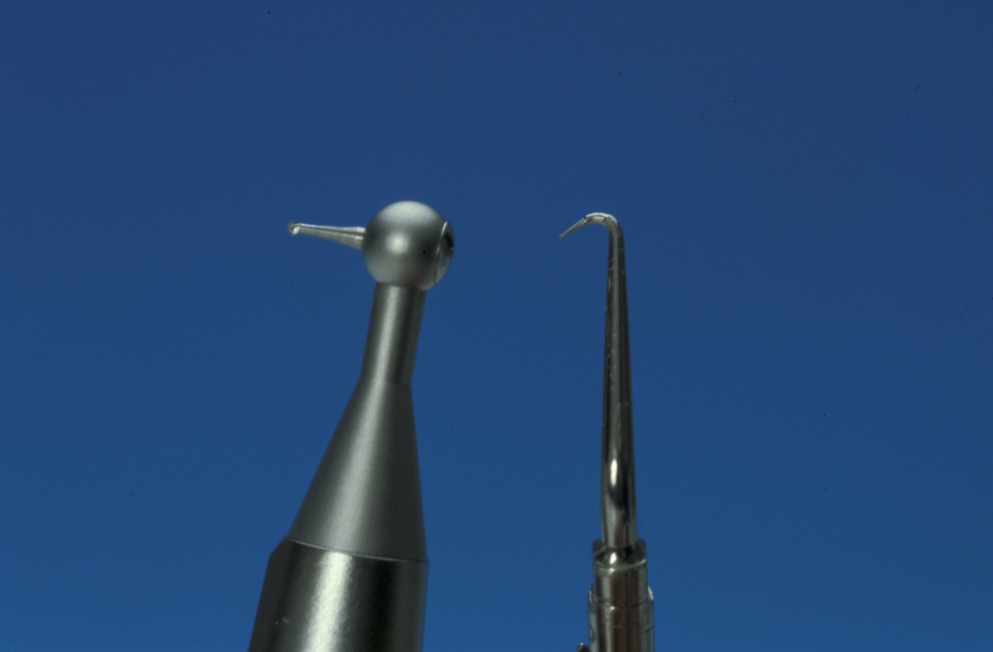
Technical details about ultrasound imaging
Sound arises from some object undergoing an oscillation, which propagates as a wave of pressure through a substance. Take an audio speaker as an example. Audio speakers contain a diaphragm which vibrates and transfers energy to the surrounding air, which causes the molecules in air to oscillate. The sound is encoded in these vibrations and is transmitted through multiple and frequent collisions between the particles in air. Figure 5 illustrates schematically particles in air undergoing compression and rarefaction (reduction in density of the air).
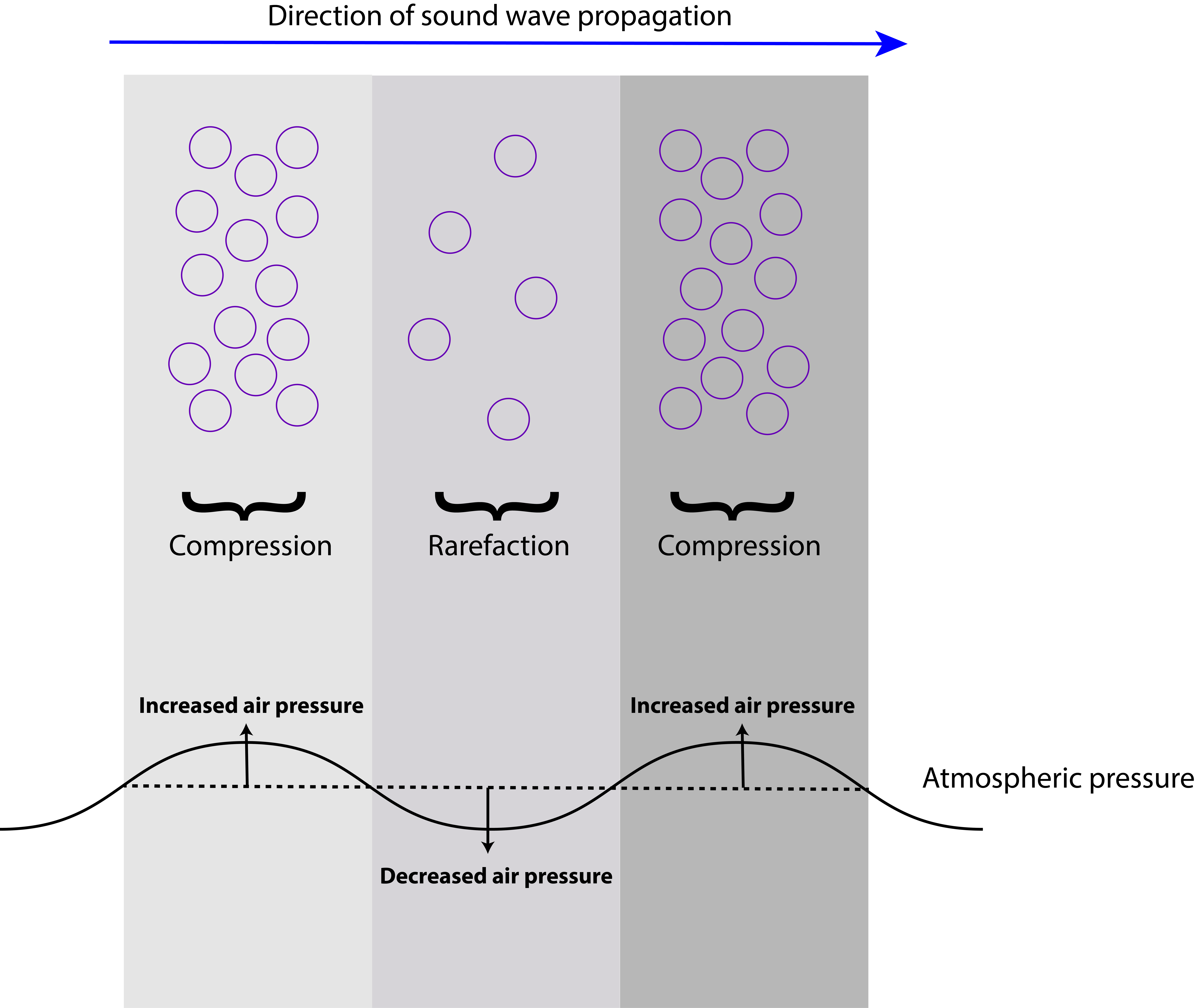
All modern ultrasound-scanning transducer probes rely on the piezoelectric effect in polar crystals to both produce the emitted ultrasound wave and to record the echo of the reflected portion of the wave. Figure 6 shows a sketch of the working principle of an ultrasound probe. Ultrasound scans of the head and neck region normally use small transducers and frequencies in the range 7.5 to 10 MHz for optimal resolution.
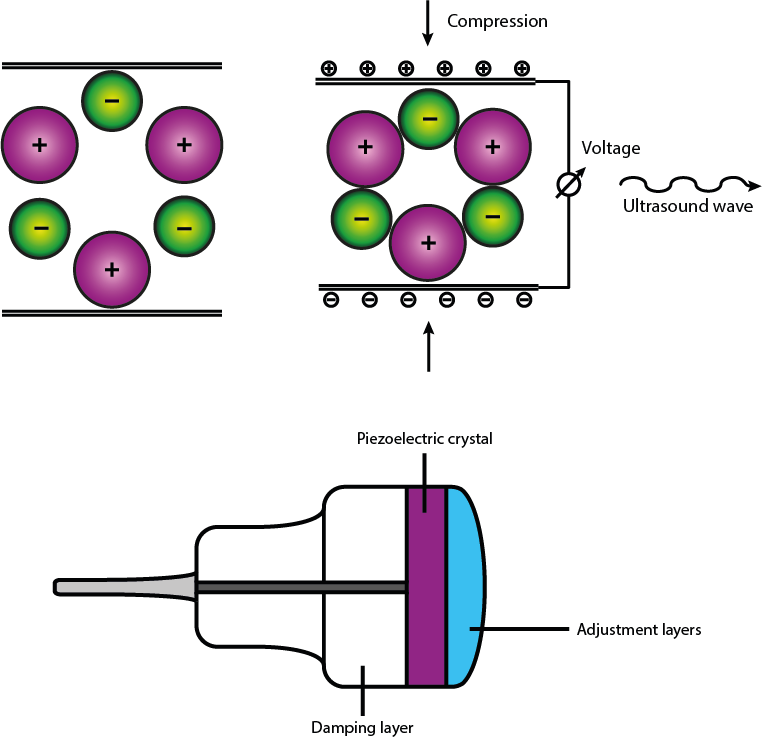
The receiver in the transducer waits for the returned echo signal and records changes in the ultrasound echo from the original emitted wave, specifically the receiver records:
- the time between the ultrasound signal being emitted and the echo being received;
- the intensity of the echo;
- the frequency of the echo;
- the direction of the echo.
Subsequently the signals are reconstructed to give an image. The vast majority of ultrasound systems focus the waves for processing via beamforming; a signal processing technique used for directional signal transmission. This formulates the ultrasound wave into an arc-shape and allows the focus to be set at a specific depth within the body.
The recorded information is fed to a central processing unit which has an array of pixels it uses to create the image. The time it takes for an echo to return determines which pixel is selected. The intensity of the echo determines the pixel brightness. Once all echoes have been processed, a greyscale image is constructed which subsequently can be displayed.
In principle, it is assumed the detected echo originates from the main ultrasound pulse and the echo returns after a single reflection. In clinical practice, this is not always the case and ultrasound imaging can be hindered by artifacts. For example, ultrasound pulses leave the transducer with a central beam and some off-axis lower energy beams called side lobes. If the side-lobe beam is reflected off a nearby surface it could be picked up by the detector. Another issue occurs when two highly reflective surfaces are close within the body; the echo may be reflected between the two surfaces multiple times before returning to the detector. This time delay would cause the central processing unit to place the echoes into pixels further away from the transducer than they are in reality. These effects can cause misleading information in scans about size and location of a scanned area / object.
Ultrasound images are relatively lower in resolution than other imaging methods. One reason for this is attenuation (see Figure 1) – the gradual loss of intensity of a wave when travelling through a medium. Attenuation causes the ultrasound wave to lose energy as it propagates, lowering the frequency of the signal. The amount of attenuation increases with higher frequencies and distances travelled. Ultrasound scanning is thus not a suitable technique to obtain high-resolution images from deep within the body.