X-ray
Contents
Effects of radiation on matter
Visible light is a type of electromagnetic radiation, but it only makes up a small part of the full spectrum (Figure 1).
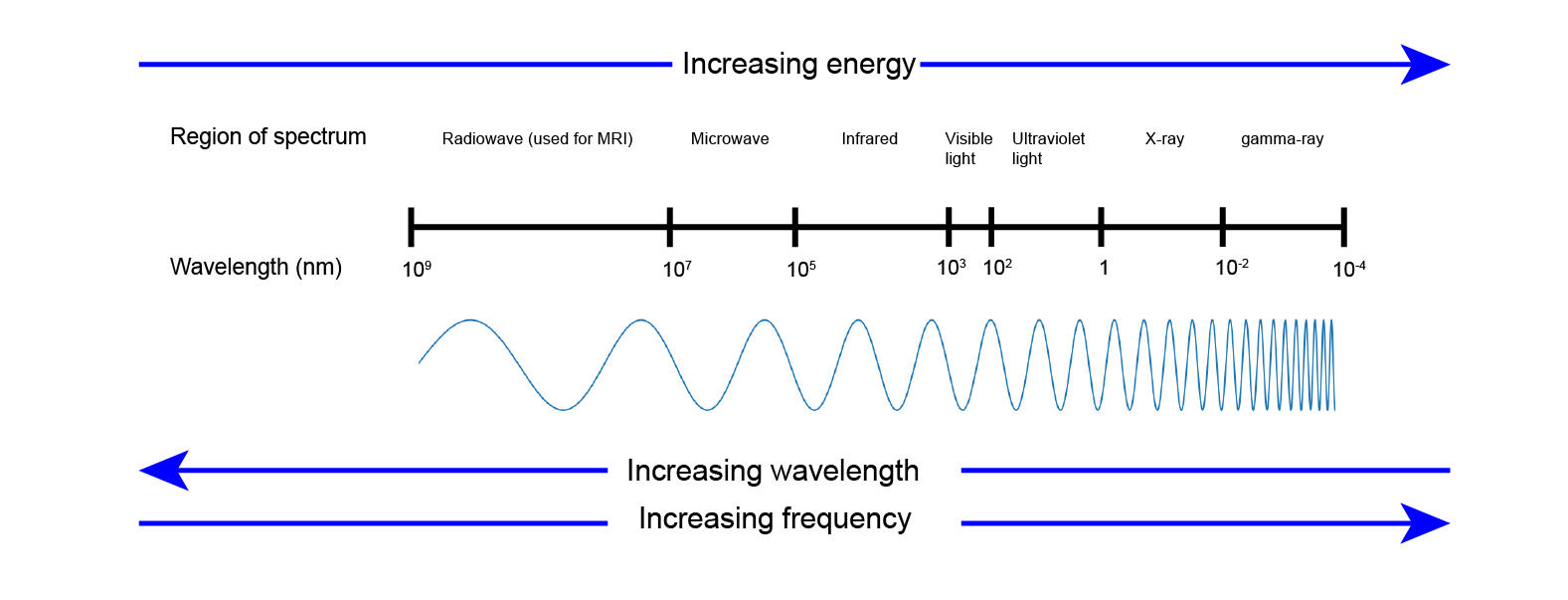
Electromagnetic radiation in the X-ray range is called ionising radiation. Its energy is high enough for it to be able to break chemical bonds in molecules and create ions as it passes through matter. X-rays interact with matter by two main processes, the photoelectric effect and Compton scattering (Figure 2). Ionisation of atoms can occur by an X-ray donating all of its energy to an electron in the photoelectric effect, or by donating some of its energy to an electron and resulting in a lower energy X-ray photon via Compton scattering.
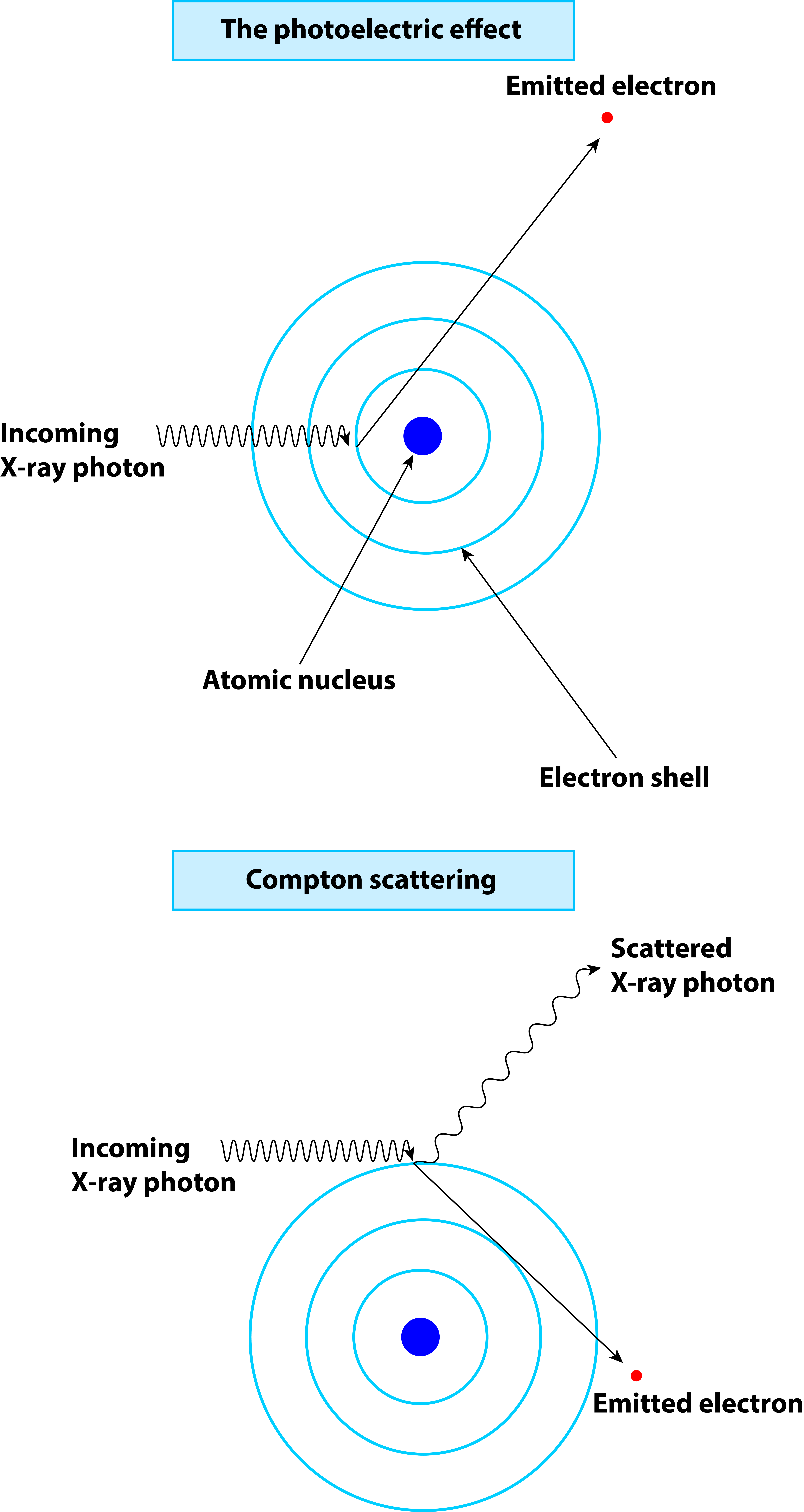
The photoelectric effect was discovered toward the end of the 19th century – experiments showed electrons could be emitted off a metallic surface when illuminated with light. In 1905, Albert Einstein explained the effect by considering electromagnetic radiation as discrete particles of energy he called photons. The energy of electromagnetic radiation is linked to its frequency (Figure 1) and if a photon of a high enough frequency strikes an electron within an atom, this photon will either be absorbed or scattered. The photoelectron effect concerns the absorption of photons. When a sufficient amount of energy is absorbed, the electron will ‘escape’ and ionise the atom.
The physicist Arthur Holly Compton suggested another effect. If Einstein’s predictions were true, if a photon’s energy was not enough to induce the photoelectric effect when colliding with an electron, the photon should recoil and be left with reduced energy. This is the principle behind the second process – Compton scattering. It results in a decrease in energy (decrease in frequency) of the photon and ionisation of the atom by emitting an electron from a peripheral electron shell.
Prolonged exposure to X-ray radiation destroys living biological tissue. Electromagnetic radiation with the energy of, or higher than, that of UV radiation can break chemical bonds between atoms in biological molecules, ionise atoms and create radicals. The mechanisms of how this damage occurs is exploited in radiotherapy with the intent to damage and destroy malignancies.
The biological effect of X-ray radiation, called the ‘biologically equivalent dose’ is often quoted in units called Sieverts (Sv). A Sv is the product of the absorbed dose of radiation and a numerical factor called the ‘relative biological effectiveness’. Relative biological effectiveness is an empirical ratio of effectiveness of one type of ionising radiation relative to another. The absorbed radiation dose is measured in Gray (Gy) and is defined as the absorption of one Joule of radiation energy per kilogram of matter (energy per unit mass; Joule (J) and kilogram (kg) are the ‘proper’ physical units).
The dosage depends on exposure time and the intensity of the beam. For all diagnostic purposes of X-ray radiation, the dosage is reduced as much as possible. For example, the exposure time is reduced by using highly sensitive detectors. The radiation intensity is reduced by filtering out the low-energy component of the X-ray spectrum using aluminium filters. This part of the X-ray radiation does not contribute to the image, so provides a dose of radiation without diagnostic information and, hence might just as well be blocked and filtered out.
The dosage levels of X-ray radiation vary widely in different applications (summarised in Table 1). The most widely used in maxillofacial diagnostic imaging in order of increasing dosage are: plain film X-ray radiographs, panoramic radiographs (OPG/DPT), cone beam computer tomographs (CBCT), and computed tomographs (CT). Even higher dosages of radiation are used in radiotherapy.
Table 1 Typical radiation dosage of common maxillofacial X-ray applications
X-Ray application | Effective dose (Gy) |
---|---|
X-ray of tooth with dental film | 0.004 |
X-ray of jaw (DPT/OPG) | 0.014 |
CBCT scan of jaw | 0.02 - 1 |
CT scan of head | 1 - 5 |
Complete course of radiotherapy to head/neck | 60 - 70 |
Creating images with X-rays
The processes by which X-ray radiation interacts with matter reduces the intensity of the ‘beam’ (attenuate the radiation) as it propagates through the body. Attenuation depends on the atomic number and density of the material that the X-ray interacts with. The more electrons an atom has, the more X-ray radiation it can absorb in either the photoelectric effect or Compton scattering (the ratio between the two effects depends on the frequency of the radiation). For example, bone is denser than soft tissue and contains atoms of higher atomic number and hence more electrons (calcium and phosphorus in bone, oxygen and carbon in soft tissue). Bone absorbs significantly more X-ray radiation energy than the equivalent thickness of soft tissue.
Images created with X-rays exploit the way different structures absorb X-rays in different amounts. After passing through the body onto a detector, fully exposed areas appear black, whereas areas which have absorbed X-rays appear in shades of grey up to white, based on how much radiation was absorbed. The variation in darkness between areas of the image is the contrast. Bones readily absorb X-rays and appear white upon the detector producing a high contrast. Conversely, X-rays which travel easily through less dense tissue such as fat and muscle, appear as shades of grey. Different types of soft tissues can be differentiated better by using contrast agents. Contrast agents in diagnostic X-ray imaging exploit the strong absorbance of X-ray radiation by heavy elements.
X-ray imaging typically makes use of collimators. These are devices that filter the X-rays so that only those travelling parallel to a specific direction can pass through the object being investigated. Collimators are a means to control the exposure to radiation. Collimators are rated by how many times they can reduce undesired radiation by half. Collimators are also used in radiotherapy treatments (intensity-modulated radiotherapy, IMRT) to shape the beam of radiation and, in consequence the irradiated area.
Photographic film is not sensitive to X-ray radiation but the results of an X-ray investigation need to be ‘translated’ to some form of visible image. Photographic film is a polymeric material, coated with an emulsion of silver salts (silver halides, typically silver chloride or bromide) suspended in gelatin. Silver salts are photosensitive and react to produce metallic silver when light falls onto them. Unmodified silver halides are sensitive only to the blue part of the visible-light spectrum. A long exposure time would be required for a well-exposed image on a photographic film when using X-ray radiation. However, increasing the exposure times increases the X-ray dosage, which is undesirable. Instead, an intensifying screen is used. The photographic film is positioned between two layers of a luminescent material (such as calcium tungstate) which readily absorbs X-rays. Calcium tungstate then emits blue light, which is detected by the photographic film (Figure 3).
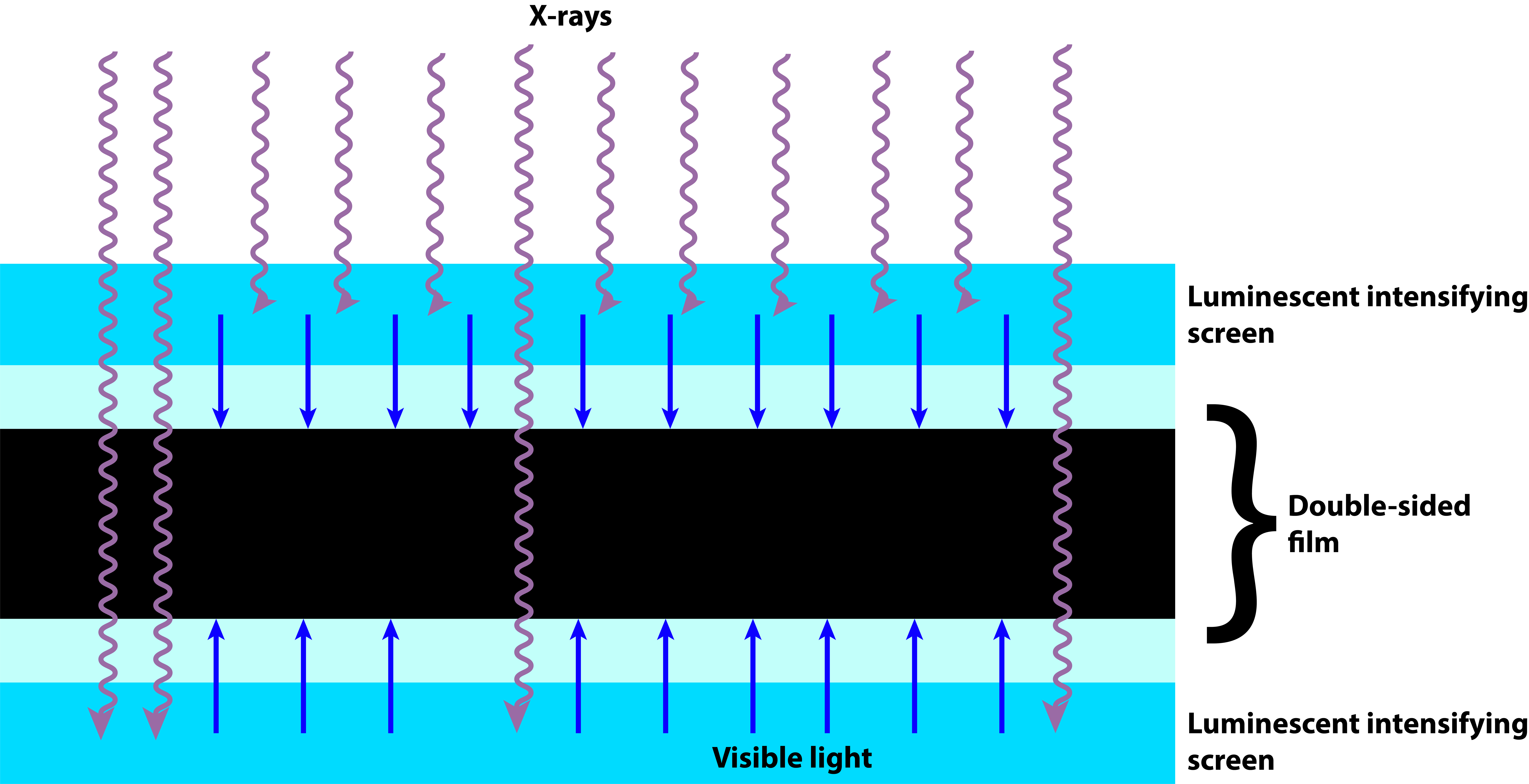
Modern X-ray images are often generated digitally with electronic detectors rather than using film. Digital recording of X-ray images has some advantages as to some extent the digital images can be processed for optimised contrast without increasing the radiation dose. A so-called image intensifier is used to record the image. X-rays strike a luminescent material which then emits light. The light then propagates to a photocathode which releases electrons. The electrons are focussed in a vacuum with electrodes and accelerate across a vacuum tube before they strike a fluorescent screen. This produces a visible image.
Some technical details of various X-ray imaging approaches
Plain film X-ray (Figure 4) gives a two-dimensional, superimposed view of a three-dimensional object. Plain film X-ray images are the simplest medical images and are the X-ray modality with the lowest exposure to X-ray radiation dosage.
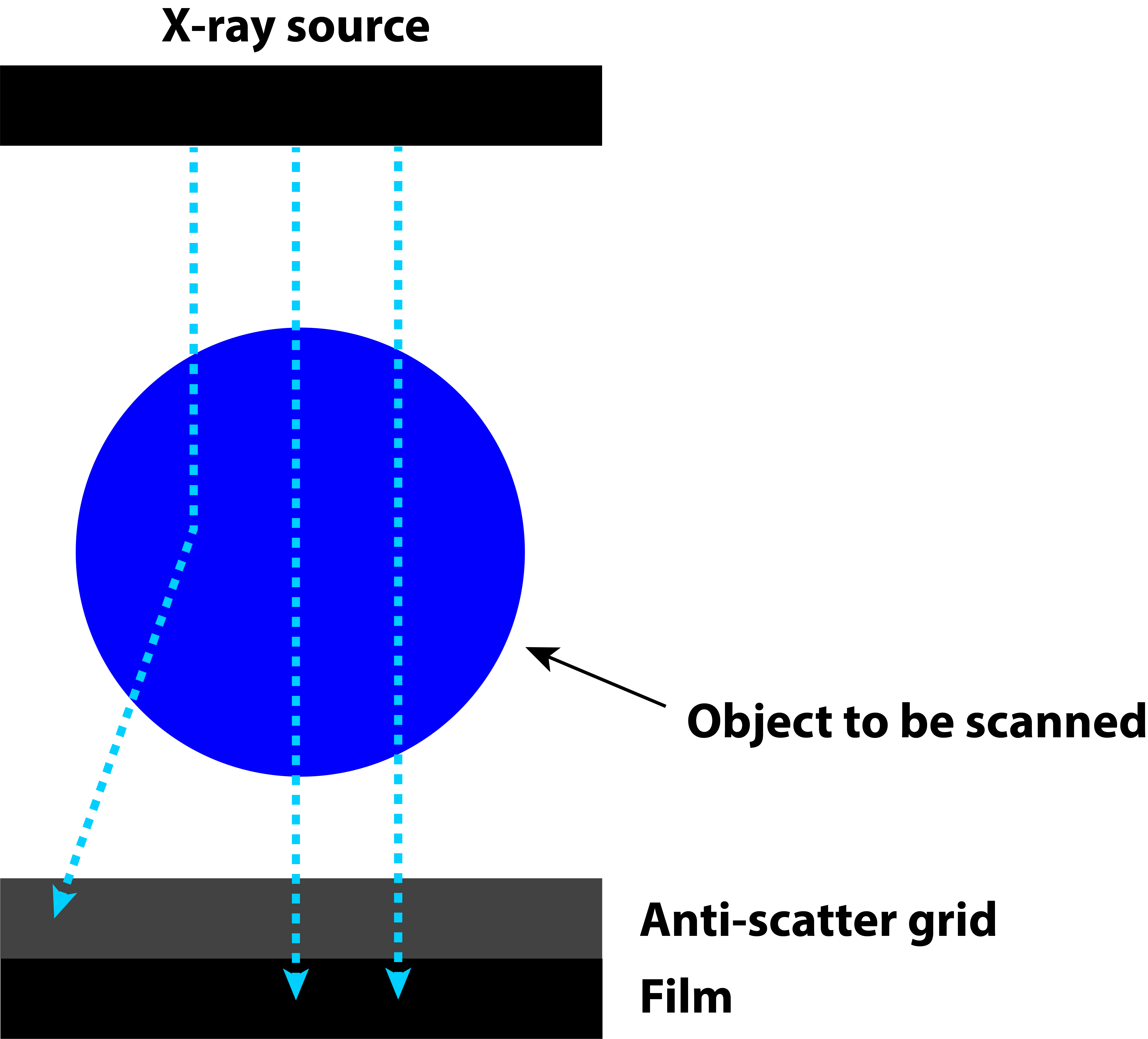
A beam of X-rays is passed through the part of the body to be scanned and recorded on an X-ray sensitive film or photographic film positioned between two luminescent layers. This simple procedure provides fast, high-resolution images of small volumes and is relatively inexpensive. The average examination for plain film X-ray images takes only a few seconds – only one snapshot image is taken.
Typical applications of plain film X-ray imaging include the identification of ectopic teeth together with dental panoramic radiographs (DPT; see below); thousands of such images are being taken daily for this purpose. Other applications of intraoral plain films include the identification of caries (bitewing radiographs), periapical pathology (at the apex of a tooth root), or to assess the viability of dental implants. Intraoral placement of a plain film X-ray holder may be impossible for somebody suffering from trismus or may be difficult in some other circumstances (such as in the presence of mucositis or other mouth ulcers). In such cases, the recording of a panoramic radiograph (DPT) may be preferable (see below).
In the recording of a panoramic radiograph (DPT), the X-ray tube and collimator/receptor rotate around the head in opposite directions. During panoramic image acquisition a continuously moving centre of rotation allows optimum image resolution across the jaw arch (Figure 5). The method yields a two-dimensional image of a three-dimensional object and enables the imaging of a larger area than plain film X-ray imaging does. The X-ray radiation dosage for the recording of a DPT is still relatively low.
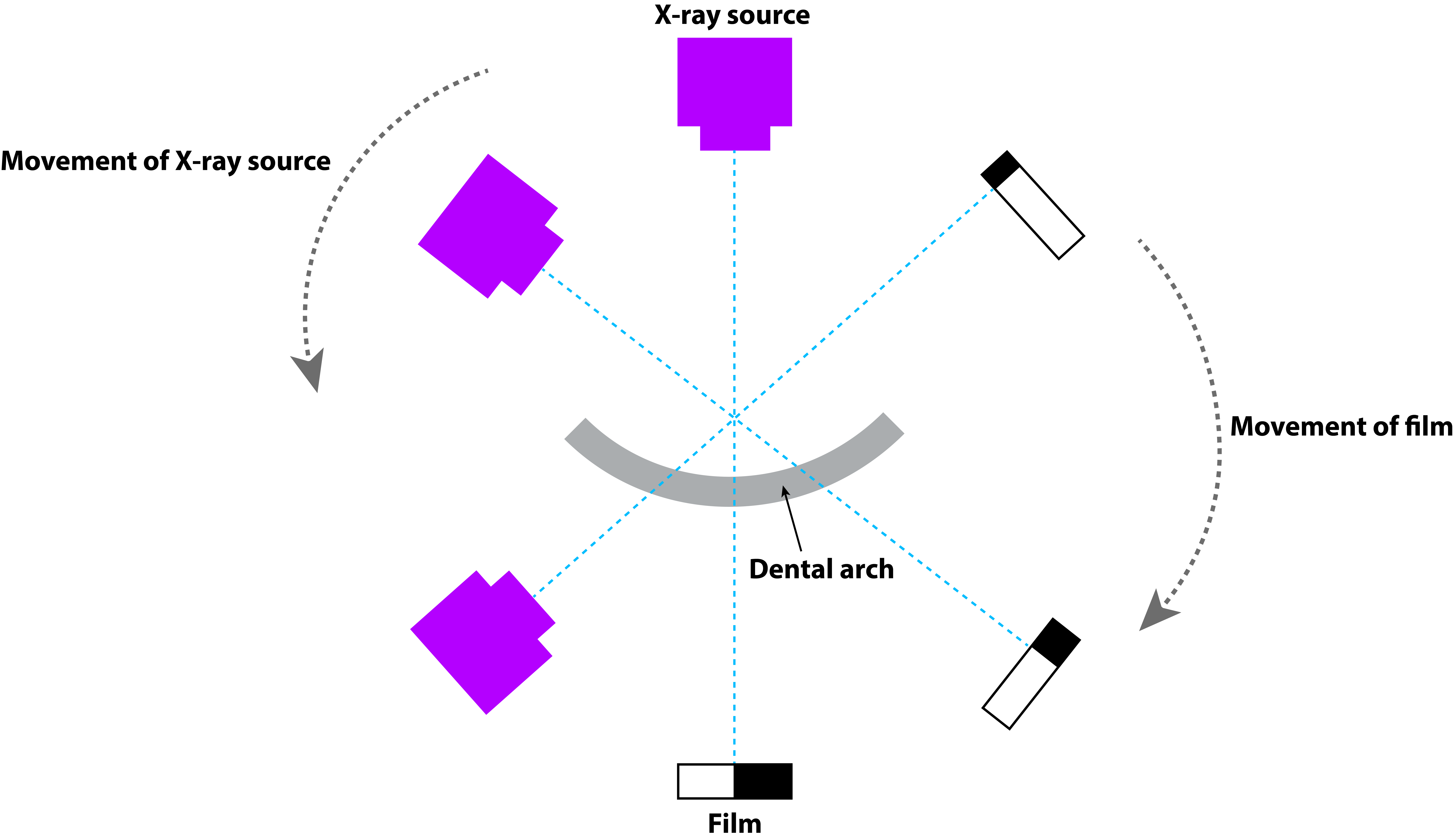
In a DPT, only structures along the dental arch are in focus. The whole process of recoding takes about one minute, with a variable radiation exposure depending on the movement of the radiation source around the head.
It is difficult to determine the precise location of objects in a DPT. Such panoramic radiographs are susceptible to errors such as distortion and double images. Image distortion is inherent to the technique because the vertical and horizontal zooms both vary differently across the image. Due the upward angle of the X-ray beam, structures closer to the X-ray source are projected higher on the image receptor than structures further away. Ghost images originate from objects present between the X-ray source and the centre of rotation (Figure 5). Ghost images appear on opposite sides of the image and higher on the image than their actual anatomical location. As they are not within the focal range of the X-ray beam, they appear blurred and magnified. Double images of objects can also occur if the object is posterior to the centre of rotation and so is struck by the beam twice. Despite all these apparent flaws, DPT is an incredibly commonly used clinical investigation in maxillofacial surgery.
The incorrect apparent location of an object in a panoramic radiograph, inherent to the creation of a two-dimensional image of a three-dimensional object is called the parallax effect. It can be overcome by exploiting the rules of geometry and projection; by simply recording a second radiograph from a different angle. The two images combined allow the determination of the correct position of an object (Figure 6). This approach has been widely used in orthodontic applications; it is capable of delivering reliable geometric information with a minimum exposure to X-ray radiation (which is highly desirable, given that orthodontic treatments are predominantly a domain of treating children and teenagers).

Dental panoramic radiographs are a workhorse in maxillofacial and oral surgery, with inferior image resolution to plain film radiographs. They are used routinely in dentoalveolar surgery, traumatology, oncology and there are those who would argue that they have a role in the complete examination of any new maxillofacial patient as DPTs can uncover pathology simply and cheaply that may not be revealed by clinical examination.
Cone beam computed tomography (CBCT) uses a divergent X-ray beam which is formed into a cone shape. A region of interest is scanned by this conically shaped X-ray beam around the vertical axis of the head (see Figure 7). It is a relative newcomer on the scene of oral and maxillofacial X-ray imaging tools.
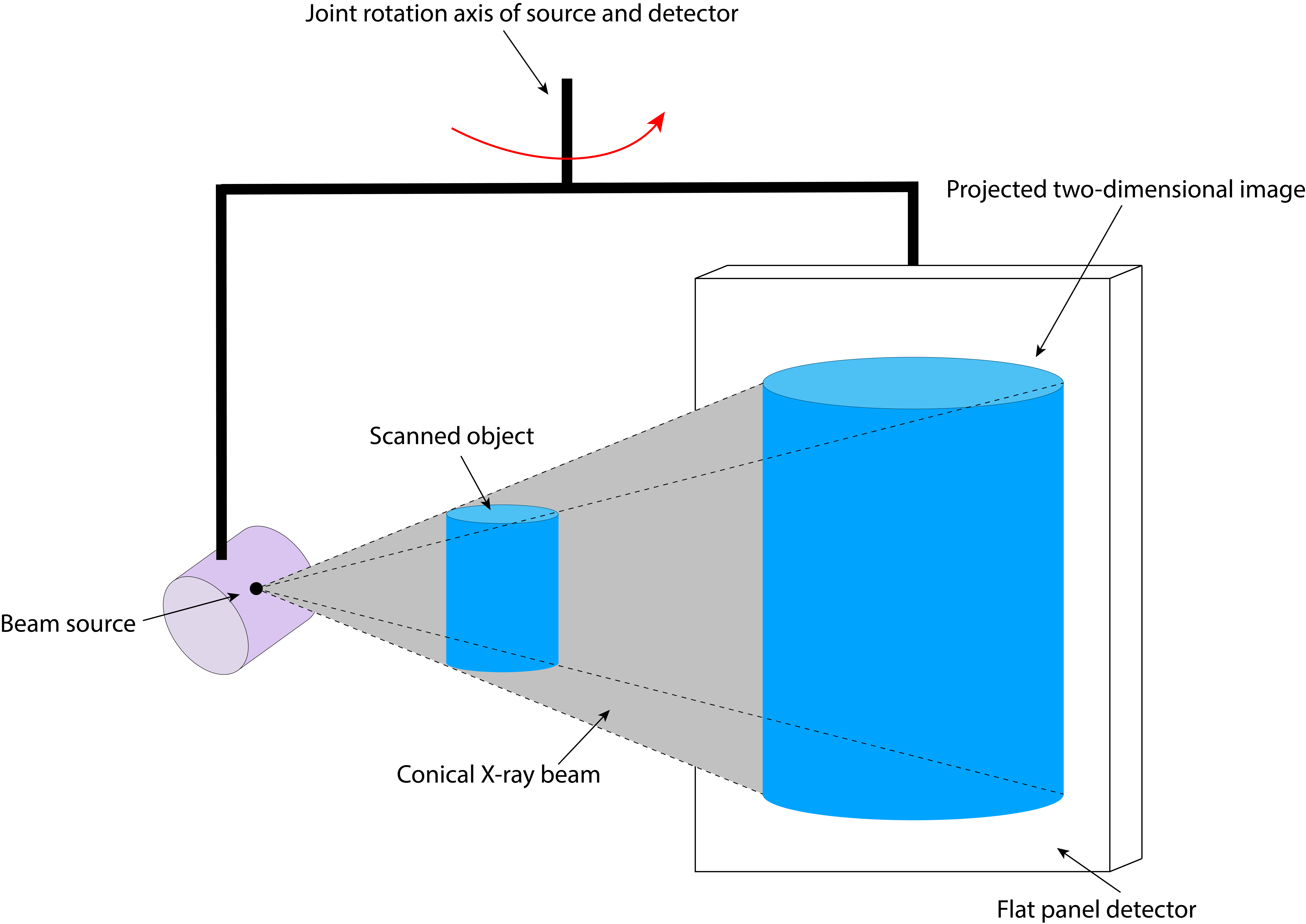
The tube and detector perform a 180 ° or 360 ° rotation round the region of interest. During rotation, the scan can obtain up to 600 distinct images. This data can be converted into arrays of data ‘slices’ which can be viewed in defined planes. The CBCT volume consists of a three-dimensional array of such image elements.
A CBCT scan carries a significantly higher exposure to X-ray radiation than does the recording of a DPT. A CT scan (which carries an even higher X-ray radiation exposure) delivers a proper three-dimensional mapping of a three-dimensional object; a CBCT scan does not really achieve this faithful mapping. There are movement artefacts and image noise. Different areas appear in different grey scales, depending on their relative position rather than different densities. This inconsistent display of attenuation is difficult to detect and/or interpret in the projection images. Detailed information on soft tissues is not obtained. In addition, so-called ring artefacts (centred on the axis of rotation) critically depend on the careful calibration of the scanner.
CBCT is much advertised as the new ‘gold standard’ of X-ray imaging. Accordingly, its use has been reported in many application areas, including dental implant planning (so far the predominant application), craniofacial deformities, cysts of the jaws, periodontal disease, endodontics, osteitis (dry socket), osteomyelitis, osteonecrosis, temporomandibular joint (jaw joint) problems and several other pathologies. For none of these applications, improved treatment outcomes have been reported when compared with the use of more traditional (two-dimensional) DPT and plain film imaging approaches. This raises the question of balance between potential benefits of having ‘a little more than two-dimensional’ information and a five- to seven-fold increased X-ray radiation exposure in comparison with DPT-based X-ray methods.
This is currently being debated and a final answer as to the merits of (or genuine indications for) CBCT is not clear. However, the following two points are clear:
- where artefacts are not acceptable (such as for surgical planning, for production of cutting templates and similar in reconstructive surgery), CT scanning remains the gold standard for obtaining a faithful three-dimensional representation;
- where artefacts are not acceptable and where hard and/or soft tissue imaging is required, MRI remains the gold standard imaging approach with much better image quality for soft tissues.